Superconducting hydrides on a quantum landscape
Abstract
Reaching superconductivity at ambient conditions is one of the biggest scientific dreams. The discoveries in the last few years at high pressures place hydrogen-based compounds as the best candidates for making it true. As the recent history shows, first-principles calculations are expected to continue guiding the experimental quest in the right track in the coming years. Considering that ionic quantum fluctuations largely affect the structural and vibrational properties of superconducting hydrides, in many cases making them stable at much lower pressures than expected, it will be crucial to include such effects on the future ab initio predictions. The prospects for low-pressure high critical-temperature compounds are wide open, even at ambient pressure.
A tale of success
The discovery of superconductivity at record temperatures in pressurized hydrogen-based compounds is one of the main triumphs of theoretical and experimental physics in the last decades. The era of superconducting hydrides sparked in 2015 after the discovery of a critical temperature () of 203 K at 155 GPa in H3S Drozdov2015 . Since then superconductivity has been reported above 200 K at megabar pressures in lanthanum PhysRevLett.122.027001 ; Drozdov2019 , yttrium PhysRevLett.126.117003 ; https://doi.org/10.1002/adma.202006832 ; Kong2021 , and calcium ma2021hightc hydrogen binary compounds, as well as in C-S-H Snider2020 and La-Y-H SEMENOK202118 ternary systems. The list of superconducting hydrides continues growing, with experimental discoveries at lower but still remarkable temperatures in Th SEMENOK202036 , Pr doi:10.1126/sciadv.aax6849 , Ce PhysRevLett.127.117001 , Ba Chen2021 , Sc, and Lu doi:10.1021/acs.inorgchem.1c01960 hydrides. All these discoveries owe a great deal to the stunning developments in diamond anvil cell (DAC) techniques, which have made possible the synthesis of new hydrogen-rich compounds at high pressures and, at the same time, the study their structural and superconducting properties mainly by x-ray diffraction and resistivity measurements Flores-Livas2020 ; doi:10.1146/annurev-conmatphys-031218-013413 .
Theoretical ab initio methods also share a big part of this success story. Many of the experimental discoveries were anticipated by first-principles calculations Duan2014 ; PhysRevLett.119.107001 ; Liu6990 ; Wang24042012 , which predicted the thermodynamical stability at high pressures of these high- compounds. These calculations apply modern ab initio crystal structure prediction techniques based on density-functional theory (DFT) Flores-Livas2020 ; Ma_NatRevMaterials_2017 ; Review_Oganov-Pickard_2019 . The quest for superconducting hydrides was launched in 2004 when Ashcroft proposed that hydrogen dominant compounds may metallize and superconduct at much lower pressures than hydrogen PhysRevLett.92.187002 . Even if the first predictions right after did not yield any extraordinary PhysRevLett.97.045504 ; gao:107002 ; PhysRevB.76.144114 ; PhysRevLett.100.045504 , the persistence of some groups finally produced predictions with critical temperatures above 200 K Wang24042012 . Now hundreds of superconducting hydrogen-rich compounds have been predicted to be thermodynamically stable at high pressures Flores-Livas2020 ; Bi2019 , with critical temperatures ranging from few kelvins to values even above 450 K PhysRevLett.123.097001 .
With the understanding that the key to enhance in hydrogen-rich compounds is the creation of electronic bonding networks and a large contribution of hydrogen to the density of states at the Fermi level belli2021strong , ab initio calculations are expected to continue predicting new high-temperature systems, specially among ternary compounds, which still remain largely unexplored. Experimental groups will be guided, hopefully in the right direction, by these calculations and new discoveries are awaited in the coming years.
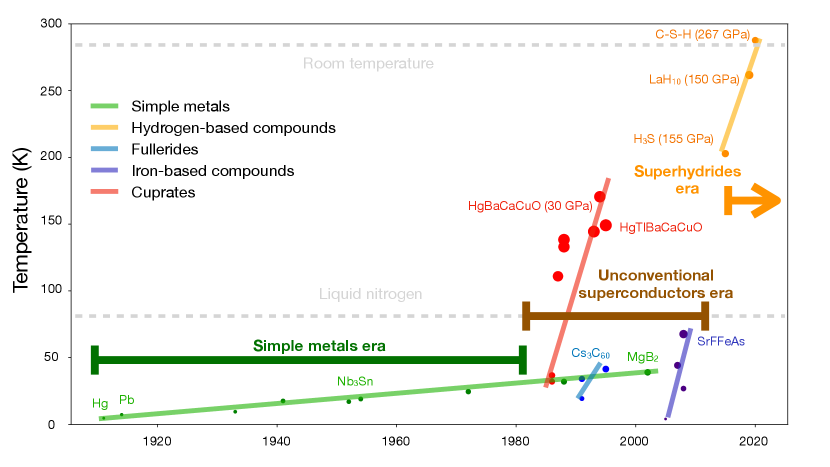
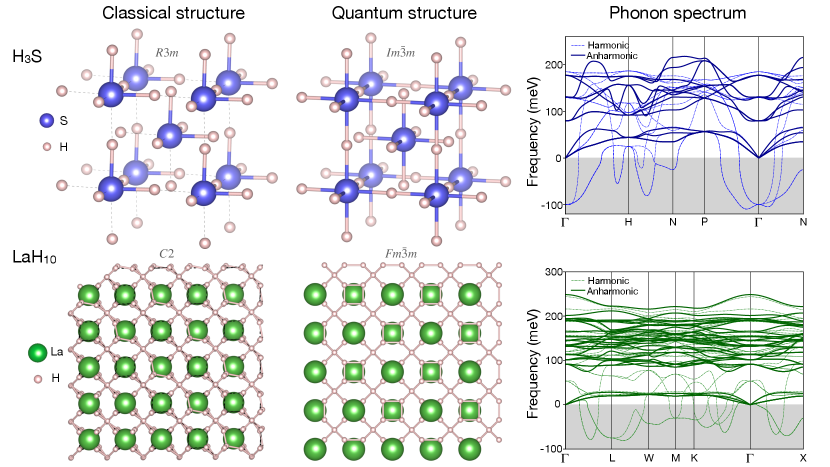
The ultimate goal
As illustrated in Fig. 1, hydrogen-rich compounds have beaten all the records set by the non-conventional cuprate superconductors in the early nineties. The possibility of room temperature superconductivity is real, but the problem is that this might only be true at high pressures. A good measure of the validity of a superconductor is the figure of merit introduced in Ref. doi:10.1146/annurev-conmatphys-031218-013413 :
(1) |
The value of of a given compound with critical temperature (in kelvin) is weighted by the pressure (in GPa) at which it superconducts, in comparison with the of MgB2 ( 39 K). Despite their large critical temperatures, the figure of merit of hydrogen-based compounds is considerably smaller than the one of the cuprates (see Fig. 1), illustrating their lack of practical applications. Hydrogen-based superconductors will be useful only if they can keep their extraordinary superconducting features at ambient pressure. This poses the ultimate goal in the field and the question to be answered by the community in the coming years: is there any high- compound thermodynamically stable or, at least, metastable at room pressure?
There are some recent interesting theoretical predictions suggesting that there might be ternary compounds with values around 100 K metastable at pressures approaching ambient. LaBH8 was predicted to be metastable above 40 GPa with a critical temperature of around 120 K PhysRevB.104.L020511 ; PhysRevB.104.134501 , while BaSiH8 and SrSiH8 have just been identified as potentially metastable as low as at 3 GPa and 27 GPa, respectively, with also high critical temperatures lucrezi2021insilico . The hope that metastable high- phases exist at ambient pressure is thus realistic, provided that the right combination of chemical elements is found.
A quantum energy landscape
The dream of ambient pressure high- superconducting hydrides is further supported by the recent ab initio calculations that show the crucial role of quantum ionic fluctuations in the stability of H3S Errea81 and LaH10 Errea66 , both with values above 200 K Drozdov2015 ; PhysRevLett.122.027001 ; Drozdov2019 . The quantum nature of the ions, which implies that due to Heisenberg’s uncertainty principle ions cannot can sit steadily in a fixed lattice site and must fluctuate around it even at 0 kelvin, stabilizes the crystal structure of the phase with the highest ’s for both compounds. In fact, the high-symmetry phase that yields the record superconductivity in LaH10 at 250 K Drozdov2019 is stable thanks to quantum effects at least 100 GPa below the pressure at which it becomes unstable within calculations that treat the ions classically, as if they were balls that stay fixed at well-defined positions Errea66 . If quantum effects can stabilize high- compounds at low pressures, they become a crucial ally in the quest for ambient pressure superconducting hydrides with remarkable critical temperatures.
The difference between classical and quantum calculations is that in the former the stable and metastable crystal structures are determined from the global and local minima of the Born-Oppenheimer energy surface , while in the latter from the minima of the quantum energy
(2) |
represents the positions of all ions in the crystal, is the kinetic energy operator of the ions, and the ionic wave function. We assume that the ionic wave function can be parametrized by centroid positions that determine the most probable ionic sites, i.e., the expectation value of the position operator, , which would correspond to the lattice sites observed experimentally.
Fig. 2 illustrates the different crystal structures obtained classically and considering quantum effects both in H3S and LaH10. The classical ground state in H3S below 175 GPa is a structure with symmetry containing asymmetric SHH bonds. Quantum effects symmetrize the bond making H atoms sit exactly halfway between two sulfur atoms, forming a structure with symmetry Errea81 , and keep it stable down to approximately 100 GPa PhysRevB.97.214101 . Ionic quantum effects also symmetrize the structure obtained classically for LaH10, with space group , to a high-symmetry phase from around 230 GPa down to at least 126 GPa. While the classical structure consists of an irregular low-symmetry network of hydrogen atoms, the quantum shows a fully symmetric clathrate structure. Remarkably, at the pressures at which these compounds reach the highest ’s the symmetric structures are identified by x-ray diffraction, not the distorted classical ones Drozdov2019 ; Einaga2016 .
The importance of ionic quantum fluctuations can be ascribed trivially to the presence of hydrogen in the system, the lightest atom in the periodic table and, consequently, the one which is more prone to quantum fluctuations. Several other hydrogen-rich compounds https://doi.org/10.1002/adma.202006832 ; PhysRevLett.111.177002 ; PhysRevB.89.064302 ; PhysRevB.103.134305 ; doi:10.1063/5.0063968 and solid hydrogen itself 0953-8984-28-49-494001 ; Monacelli2021Black are also largely affected by such effects. There is, however, another less apparent but very important point that makes ionic quantum effects crucial in high- superconducting hydrides: their huge electron-phonon coupling induces large phonon instabilities in the harmonic approximation. Indeed, Allen and Cohen argued long time ago how a large electron-phonon interaction can soften phonon frequencies and eventually drive a system dynamically unstable PhysRevLett.29.1593 . This argument is only valid for harmonic phonons derived from the Hessian of the Born-Oppenheimer potential. In fact, as illustrated in Fig. 2, the harmonic phonon frequencies of H3S and LaH10 in the high-symmetry phases have large phonon instabilities, identified as negative eigenvalues of the Hessian, that underline that these structures are not local minima of the potential. However, in the quantum world ions live in, the stability of a structure has to be determined instead by the eigenvalues of the Hessian of the quantum energy. Fig. 2 shows that these are indeed positive, so that the high symmetry phases are local (and also global) minima of the quantum energy. As in the calculation of all terms of the potential contribute (see Eq. (2)), the latter is essentially an anharmonic result. Since high-order anharmonic terms of the Born-Oppenheimer potential will become important whenever the second-order harmonic terms are small, such as when harmonic phonons are softened, hydrides with large electron-phonon coupling are expected to be largely affected by anharmonicity.
A complex calculation
Calculating structural and vibrational properties considering ionic quantum effects and the consequent anharmonicity is not a simple task for ab initio methods, mainly due to the non-perturbative treatment that anharmonicity requires. In fact, in situations like those sketched in the phonon spectra of Fig. 2 for the high-symmetry phases of H3S and LaH10 no perturbative treatment of anharmonicity is possible: the harmonic approximation has no ground state upon which a perturbative expansion can be built due to the presence of imaginary phonons.
Despite being intrinsically non-perturbative, since the ion’s dynamics are based on classical Newtonian mechanics, widely spread ab initio molecular dynamic (AIMD) methods PhysRevLett.55.2471 fail capturing these ionic quantum effects. Phonon frequencies with the largest contribution to the electron-phonon coupling have energies of around 100 meV, which means that the ionic displacements associated to them remain essentially quantum mechanical even at temperatures as high as 1000 K. A valid alternative is offered by path integral molecular dynamics (PIMD) simulations RevModPhys.67.279 , which incorporate the quantum mechanical motion of ions at the prize of increasing the computational cost in approximately one order of magnitude. A systematic use of PIMD methods is also hindered by the difficulties of extracting phonon frequencies and identifying the equilibrium crystal structures without performing very long, and thus expensive, simulations.
A computationally less expensive alternative, which can determine the structures at the minima of the quantum energy and yield phonon spectra considering anharmonicity non-perturbatively, is offered by the stochastic self-consistent harmonic approximation (SSCHA) PhysRevB.89.064302 ; PhysRevB.96.014111 ; PhysRevB.98.024106 ; Monacelli_2021 . The idea of this method is to parametrize the ionic wave functions with Gaussians centered at centroid positions , with a width determined by some effective force constants . By calculating the quantum energy in Eq. (2) and minimizing it with respect to and , the stable and metastable phases in the quantum energy landscape can be determined, while the phonon frequencies can be determined from the Hessian of the energy. This method has led correctly to the experimentally observed crystal structures in high- H3S and LaH10 Errea81 ; Errea66 . Including the SSCHA phonons in the calculations of the critical temperature within Migdal-Eliashberg theory, values in agreement with experiments have been reached as well Errea81 ; Errea66 ; PhysRevLett.114.157004 . This method has also helped to comprehend the different experimental interpretations of the metallization of high-pressure hydrogen Monacelli2021Black ; Dias715 ; Eremets2019 ; Loubeyre2020 .
Can we make crystal structure predictions on the quantum energy landscape?
The challenge for the next years is to perform crystal structure predictions on the quantum energy landscape. All crystal structures predictions performed so far work instead on the classical Born-Oppenherimer energy surface. It is true that these classical calculations have been able to predict high- hydrides Duan2014 ; PhysRevLett.119.107001 ; Liu6990 ; Wang24042012 , but generally the high-symmetry and high- structures are found at much higher pressures than observed later experimentally due to the instabilities appearing at low pressures. If we were able to perform crystal structure predictions directly on the quantum energy landscape, we would be able possibly to predict high- compounds at low pressures that are currently missed by approaches not considering ionic quantum fluctuations.
The problem is that this approach requires in principle a PIMD or SSCHA calculation for each of the hundreds, or even thousands, of compounds generated in the crystal structure prediction process. This is a daunting task, not feasible with current computational resources. Discarding such an unrealistic approach, it seems more appropriate to relax in the quantum energy landscape just the most competitive phases found classically. This approach will be valid if the structures which are local minima of the classical potential are not far from those that are minima of , in the sense that starting, for instance, a SSCHA relaxation from the classical structures the quantum structures are obtained, like in the LaH10 case Errea66 .
The computational difficulties arise if the sampling of the Born-Oppenheimer potential needed to evaluate Eq. (2) is performed within DFT. As mentioned above, DFT-based crystal strucuture predictions with semi-local functionals PhysRevLett.77.3865 have turned out to be very successful. However, whether a DFT sampling is actually necessary is not clear. Could machine-learned potentials Deringer2021 alternatively be used for the quantum calculations? This would definitely speed up the quantum calculations and, provided a good potential is obtained to describe the interactions of the system, it may be possible to make structural predictions on the quantum energy landscape directly. Despite machine-learned potentials are rather sensitive to the local environment, which limits their transferability, it is encouraging that a machine learned potential has been able to describe the polymorphism of solid hydrogen at high pressures as well as its molecular to atomic liquid transition Cheng2020 . One can dream of obtaining a machine learned potential with DFT accuracy by fitting it to the forces obtained during the classical structure prediction, and later use it for the quantum relaxations also at other thermodynamic conditions.
New discoveries to come
Considering that classical algorithms already predict metastable superconducting ternary compounds at low pressures and that ionic quantum effects seem to decrease the stabilization pressure of high- structures, it is reasonable to have faith on new exciting discoveries to come in the following years. The field is not at all exhausted, and the incorporation of ionic quantum effects into state-of-the-art crystal structure prediction methods will be crucial to guide experimentalists into new superconducting hydrides with a large figure of merit. Indeed, superconducting hydrides live on a quantum landscape.
Acknowledgements
This work was supported by the European Research Council (ERC) under the European Unions Horizon 2020 research and innovation programme (grant agreement No. 802533).
References
- (1) A. P. Drozdov, M. I. Eremets, I. A. Troyan, V. Ksenofontov, and S. I. Shylin. Conventional superconductivity at 203 kelvin at high pressures in the sulfur hydride system. Nature, 525(7567):73–76, Sep 2015.
- (2) Maddury Somayazulu, Muhtar Ahart, Ajay K. Mishra, Zachary M. Geballe, Maria Baldini, Yue Meng, Viktor V. Struzhkin, and Russell J. Hemley. Evidence for superconductivity above 260 k in lanthanum superhydride at megabar pressures. Phys. Rev. Lett., 122:027001, Jan 2019.
- (3) A. P. Drozdov, P. P. Kong, V. S. Minkov, S. P. Besedin, M. A. Kuzovnikov, S. Mozaffari, L. Balicas, F. F. Balakirev, D. E. Graf, V. B. Prakapenka, E. Greenberg, D. A. Knyazev, M. Tkacz, and M. I. Eremets. Superconductivity at 250 k in lanthanum hydride under high pressures. Nature, 569(7757):528–531, May 2019.
- (4) Elliot Snider, Nathan Dasenbrock-Gammon, Raymond McBride, Xiaoyu Wang, Noah Meyers, Keith V. Lawler, Eva Zurek, Ashkan Salamat, and Ranga P. Dias. Synthesis of yttrium superhydride superconductor with a transition temperature up to 262 k by catalytic hydrogenation at high pressures. Phys. Rev. Lett., 126:117003, Mar 2021.
- (5) Ivan A. Troyan, Dmitrii V. Semenok, Alexander G. Kvashnin, Andrey V. Sadakov, Oleg A. Sobolevskiy, Vladimir M. Pudalov, Anna G. Ivanova, Vitali B. Prakapenka, Eran Greenberg, Alexander G. Gavriliuk, Igor S. Lyubutin, Viktor V. Struzhkin, Aitor Bergara, Ion Errea, Raffaello Bianco, Matteo Calandra, Francesco Mauri, Lorenzo Monacelli, Ryosuke Akashi, and Artem R. Oganov. Anomalous high-temperature superconductivity in yh6. Advanced Materials, 33(15):2006832, 2021.
- (6) Panpan Kong, Vasily S. Minkov, Mikhail A. Kuzovnikov, Alexander P. Drozdov, Stanislav P. Besedin, Shirin Mozaffari, Luis Balicas, Fedor Fedorovich Balakirev, Vitali B. Prakapenka, Stella Chariton, Dmitry A. Knyazev, Eran Greenberg, and Mikhail I. Eremets. Superconductivity up to 243 k in the yttrium-hydrogen system under high pressure. Nature Communications, 12(1):5075, Aug 2021.
- (7) Liang Ma, Kui Wang, Yu Xie, Xin Yang, Yingying Wang, Mi Zhou, Hanyu Liu, Xiaohui Yu, Yongsheng Zhao, Hongbo Wang, Guangtao Liu, and Yanming Ma. High-tc superconductivity in clathrate calcium hydride cah6. arXiv, page 2103.16282, 2021.
- (8) Elliot Snider, Nathan Dasenbrock-Gammon, Raymond McBride, Mathew Debessai, Hiranya Vindana, Kevin Vencatasamy, Keith V. Lawler, Ashkan Salamat, and Ranga P. Dias. Room-temperature superconductivity in a carbonaceous sulfur hydride. Nature, 586(7829):373–377, Oct 2020.
- (9) Dmitrii V. Semenok, Ivan A. Troyan, Anna G. Ivanova, Alexander G. Kvashnin, Ivan A. Kruglov, Michael Hanfland, Andrey V. Sadakov, Oleg A. Sobolevskiy, Kirill S. Pervakov, Igor S. Lyubutin, Konstantin V. Glazyrin, Nico Giordano, Denis N. Karimov, Alexander L. Vasiliev, Ryosuke Akashi, Vladimir M. Pudalov, and Artem R. Oganov. Superconductivity at 253 k in lanthanum–yttrium ternary hydrides. Materials Today, 48:18–28, 2021.
- (10) Dmitry V. Semenok, Alexander G. Kvashnin, Anna G. Ivanova, Volodymyr Svitlyk, Vyacheslav Yu. Fominski, Andrey V. Sadakov, Oleg A. Sobolevskiy, Vladimir M. Pudalov, Ivan A. Troyan, and Artem R. Oganov. Superconductivity at 161 k in thorium hydride thh10: Synthesis and properties. Materials Today, 33:36–44, 2020.
- (11) Di Zhou, Dmitrii V. Semenok, Defang Duan, Hui Xie, Wuhao Chen, Xiaoli Huang, Xin Li, Bingbing Liu, Artem R. Oganov, and Tian Cui. Superconducting praseodymium superhydrides. Science Advances, 6(9):eaax6849, 2020.
- (12) Wuhao Chen, Dmitrii V. Semenok, Xiaoli Huang, Haiyun Shu, Xin Li, Defang Duan, Tian Cui, and Artem R. Oganov. High-temperature superconducting phases in cerium superhydride with a up to 115 k below a pressure of 1 megabar. Phys. Rev. Lett., 127:117001, Sep 2021.
- (13) Wuhao Chen, Dmitrii V. Semenok, Alexander G. Kvashnin, Xiaoli Huang, Ivan A. Kruglov, Michele Galasso, Hao Song, Defang Duan, Alexander F. Goncharov, Vitali B. Prakapenka, Artem R. Oganov, and Tian Cui. Synthesis of molecular metallic barium superhydride: pseudocubic bah12. Nature Communications, 12(1):273, Jan 2021.
- (14) Mengyao Shao, Su Chen, Wuhao Chen, Kexin Zhang, Xiaoli Huang, and Tian Cui. Superconducting sch3 and luh3 at megabar pressures. Inorganic Chemistry, 60(20):15330–15335, 2021. PMID: 34590849.
- (15) José A. Flores-Livas, Lilia Boeri, Antonio Sanna, Gianni Profeta, Ryotaro Arita, and Mikhail Eremets. A perspective on conventional high-temperature superconductors at high pressure: Methods and materials. Physics Reports, 856:1 – 78, 2020. A perspective on conventional high-temperature superconductors at high pressure: Methods and materials.
- (16) Chris J. Pickard, Ion Errea, and Mikhail I. Eremets. Superconducting hydrides under pressure. Annual Review of Condensed Matter Physics, 11(1):57–76, 2020.
- (17) Defang Duan, Yunxian Liu, Fubo Tian, Da Li, Xiaoli Huang, Zhonglong Zhao, Hongyu Yu, Bingbing Liu, Wenjing Tian, and Tian Cui. Pressure-induced metallization of dense (h2s)2h2 with high-tc superconductivity. Sci. Rep., 4, Nov 2014.
- (18) Feng Peng, Ying Sun, Chris J. Pickard, Richard J. Needs, Qiang Wu, and Yanming Ma. Hydrogen clathrate structures in rare earth hydrides at high pressures: Possible route to room-temperature superconductivity. Phys. Rev. Lett., 119:107001, Sep 2017.
- (19) Hanyu Liu, Ivan I. Naumov, Roald Hoffmann, N. W. Ashcroft, and Russell J. Hemley. Potential high-tc superconducting lanthanum and yttrium hydrides at high pressure. Proceedings of the National Academy of Sciences, 114(27):6990–6995, 2017.
- (20) Hui Wang, John S. Tse, Kaori Tanaka, Toshiaki Iitaka, and Yanming Ma. Superconductive sodalite-like clathrate calcium hydride at high pressures. Proceedings of the National Academy of Sciences, 109(17):6463–6466, 2012.
- (21) Lijun Zhang, Yanchao Wang, Jian Lv, and Yanming Ma. Materials discovery at high pressures. Nature Reviews Materials, 2(4):17005, 2017.
- (22) Artem R. Oganov, Chris J. Pickard, Qiang Zhu, and Richard J. Needs. Structure prediction drives materials discovery. Nature Reviews Materials, 4(5):331–348, 2019.
- (23) N. W. Ashcroft. Hydrogen dominant metallic alloys: High temperature superconductors? Phys. Rev. Lett., 92(18):187002, May 2004.
- (24) Chris J. Pickard and R. J. Needs. High-pressure phases of silane. Phys. Rev. Lett., 97:045504, Jul 2006.
- (25) Guoying Gao, Artem R. Oganov, Aitor Bergara, Miguel Martinez-Canales, Tian Cui, Toshiaki Iitaka, Yanming Ma, and Guangtian Zou. Superconducting high pressure phase of germane. Phys. Rev. Lett., 101(10):107002, 2008.
- (26) Chris J. Pickard and R. J. Needs. Metallization of aluminum hydride at high pressures: A first-principles study. Phys. Rev. B, 76:144114, Oct 2007.
- (27) Igor Goncharenko, M. I. Eremets, M. Hanfland, J. S. Tse, M. Amboage, Y. Yao, and I. A. Trojan. Pressure-induced hydrogen-dominant metallic state in aluminum hydride. Phys. Rev. Lett., 100(4):045504, Jan 2008.
- (28) Tiange Bi, Niloofar Zarifi, Tyson Terpstra, and Eva Zurek. The search for superconductivity in high pressure hydrides. In Reference Module in Chemistry, Molecular Sciences and Chemical Engineering. Elsevier, 2019.
- (29) Ying Sun, Jian Lv, Yu Xie, Hanyu Liu, and Yanming Ma. Route to a superconducting phase above room temperature in electron-doped hydride compounds under high pressure. Phys. Rev. Lett., 123:097001, Aug 2019.
- (30) Francesco Belli, Trinidad Novoa, J. Contreras-García, and Ion Errea. Strong correlation between electronic bonding network and critical temperature in hydrogen-based superconductors. Nature Communications, 12(1):5381, Sep 2021.
- (31) Ion Errea, Matteo Calandra, Chris J. Pickard, Joseph R. Nelson, Richard J. Needs, Yinwei Li, Hanyu Liu, Yunwei Zhang, Yanming Ma, and Francesco Mauri. Quantum hydrogen-bond symmetrization in the superconducting hydrogen sulfide system. Nature, 532(7597):81–84, Apr 2016. Letter.
- (32) Ion Errea, Francesco Belli, Lorenzo Monacelli, Antonio Sanna, Takashi Koretsune, Terumasa Tadano, Raffaello Bianco, Matteo Calandra, Ryotaro Arita, Francesco Mauri, and José A. Flores-Livas. Quantum crystal structure in the 250-kelvin superconducting lanthanum hydride. Nature, 578(7793):66–69, Feb 2020.
- (33) Simone Di Cataldo, Christoph Heil, Wolfgang von der Linden, and Lilia Boeri. : Towards high- low-pressure superconductivity in ternary superhydrides. Phys. Rev. B, 104:L020511, Jul 2021.
- (34) Xiaowei Liang, Aitor Bergara, Xudong Wei, Xiaoxu Song, Linyan Wang, Rongxin Sun, Hanyu Liu, Russell J. Hemley, Lin Wang, Guoying Gao, and Yongjun Tian. Prediction of high- superconductivity in ternary lanthanum borohydrides. Phys. Rev. B, 104:134501, Oct 2021.
- (35) Roman Lucrezi, Simone Di Cataldo, Wolfgang von der Linden, Lilia Boeri, and Christoph Heil. In-silico synthesis of novel lowest-pressure high- ternary superhydrides. page arXiv:2112.02131, 2021.
- (36) Raffaello Bianco, Ion Errea, Matteo Calandra, and Francesco Mauri. High-pressure phase diagram of hydrogen and deuterium sulfides from first principles: Structural and vibrational properties including quantum and anharmonic effects. Phys. Rev. B, 97:214101, Jun 2018.
- (37) Mari Einaga, Masafumi Sakata, Takahiro Ishikawa, Katsuya Shimizu, Mikhail I. Eremets, Alexander P. Drozdov, Ivan A. Troyan, Naohisa Hirao, and Yasuo Ohishi. Crystal structure of the superconducting phase of sulfur hydride. Nature Physics, 12(9):835–838, Sep 2016.
- (38) Ion Errea, Matteo Calandra, and Francesco Mauri. First-principles theory of anharmonicity and the inverse isotope effect in superconducting palladium-hydride compounds. Phys. Rev. Lett., 111:177002, Oct 2013.
- (39) Ion Errea, Matteo Calandra, and Francesco Mauri. Anharmonic free energies and phonon dispersions from the stochastic self-consistent harmonic approximation: Application to platinum and palladium hydrides. Phys. Rev. B, 89:064302, Feb 2014.
- (40) Pugeng Hou, Francesco Belli, Raffaello Bianco, and Ion Errea. Strong anharmonic and quantum effects in under high pressure: A first-principles study. Phys. Rev. B, 103:134305, Apr 2021.
- (41) Pugeng Hou, Francesco Belli, Raffaello Bianco, and Ion Errea. Quantum anharmonic enhancement of superconductivity in p63/mmc sch6 at high pressures: A first-principles study. Journal of Applied Physics, 130(17):175902, 2021.
- (42) Miguel Borinaga, P Riego, A Leonardo, Matteo Calandra, Francesco Mauri, Aitor Bergara, and Ion Errea. Anharmonic enhancement of superconductivity in metallic molecular cmca ¿¿¿¿¿4 hydrogen at high pressure: a first-principles study. Journal of Physics: Condensed Matter, 28(49):494001, 2016.
- (43) Lorenzo Monacelli, Ion Errea, Matteo Calandra, and Francesco Mauri. Black metal hydrogen above 360 gpa driven by proton quantum fluctuations. Nature Physics, 17(1):63–67, Jan 2021.
- (44) Philip B. Allen and Marvin L. Cohen. Superconductivity and phonon softening. Phys. Rev. Lett., 29(24):1593–1596, 1972.
- (45) R. Car and M. Parrinello. Unified approach for molecular dynamics and density-functional theory. Phys. Rev. Lett., 55:2471–2474, Nov 1985.
- (46) D. M. Ceperley. Path integrals in the theory of condensed helium. Rev. Mod. Phys., 67:279–355, Apr 1995.
- (47) Raffaello Bianco, Ion Errea, Lorenzo Paulatto, Matteo Calandra, and Francesco Mauri. Second-order structural phase transitions, free energy curvature, and temperature-dependent anharmonic phonons in the self-consistent harmonic approximation: Theory and stochastic implementation. Phys. Rev. B, 96:014111, Jul 2017.
- (48) Lorenzo Monacelli, Ion Errea, Matteo Calandra, and Francesco Mauri. Pressure and stress tensor of complex anharmonic crystals within the stochastic self-consistent harmonic approximation. Phys. Rev. B, 98:024106, Jul 2018.
- (49) Lorenzo Monacelli, Raffaello Bianco, Marco Cherubini, Matteo Calandra, Ion Errea, and Francesco Mauri. The stochastic self-consistent harmonic approximation: calculating vibrational properties of materials with full quantum and anharmonic effects. Journal of Physics: Condensed Matter, 33(36):363001, jul 2021.
- (50) Ion Errea, Matteo Calandra, Chris J. Pickard, Joseph Nelson, Richard J. Needs, Yinwei Li, Hanyu Liu, Yunwei Zhang, Yanming Ma, and Francesco Mauri. High-pressure hydrogen sulfide from first principles: A strongly anharmonic phonon-mediated superconductor. Phys. Rev. Lett., 114:157004, Apr 2015.
- (51) Ranga P. Dias and Isaac F. Silvera. Observation of the wigner-huntington transition to metallic hydrogen. Science, 355(6326):715–718, 2017.
- (52) M. I. Eremets, A. P. Drozdov, P. P. Kong, and H. Wang. Semimetallic molecular hydrogen at pressure above 350 gpa. Nature Physics, 15(12):1246–1249, Dec 2019.
- (53) Paul Loubeyre, Florent Occelli, and Paul Dumas. Synchrotron infrared spectroscopic evidence of the probable transition to metal hydrogen. Nature, 577(7792):631–635, Jan 2020.
- (54) John P. Perdew, Kieron Burke, and Matthias Ernzerhof. Generalized gradient approximation made simple. Phys. Rev. Lett., 77(18):3865–3868, 1996.
- (55) Volker L. Deringer, Albert P. Bartók, Noam Bernstein, David M. Wilkins, Michele Ceriotti, and Gábor Csányi. Gaussian process regression for materials and molecules. Chemical Reviews, 121(16):10073–10141, Aug 2021.
- (56) Bingqing Cheng, Guglielmo Mazzola, Chris J. Pickard, and Michele Ceriotti. Evidence for supercritical behaviour of high-pressure liquid hydrogen. Nature, 585(7824):217–220, Sep 2020.