First high-precision direct determination of the atomic mass of a superheavy nuclide
Abstract
We present the first direct measurement of the atomic mass of a superheavy nuclide. Atoms of 257Db (=105) were produced online at the RIKEN Nishina Center for Accelerator-Based Science using the fusion-evaporation reaction 208Pb(51V, 2n)257Db. The gas-filled recoil ion separator GARIS-II was used to suppress both the unreacted primary beam and some transfer products, prior to delivering the energetic beam of 257Db ions to a helium gas-filled ion stopping cell wherein they were thermalized. Thermalized 257Db3+ ions were then transferred to a multi-reflection time-of-flight mass spectrograph for mass analysis. An alpha particle detector embedded in the ion time-of-flight detector allowed disambiguation of the rare 257Db3+ time-of-flight detection events from background by means of correlation with characteristic -decays. The extreme sensitivity of this technique allowed a precision atomic mass determination from 11 events. The mass excess was determined to be keV/c2. Comparing to several mass models, we show the technique can be used to unambiguously determine the atomic number as =105 and should allow similar evaluations for heavier species in future work.
pacs:
Valid PACS appear hereThe unambiguous identification of superheavy nuclei is a longstanding issue that has largely been achieved through cross-bombardment experiments in recent years Oganessian2013 ; Oganessian2004 ; MoritaZ113 . For the hot fusion superheavy nuclides (SHN) located “northeast” of 263Rf, however, cross-bombardment does not fully resolve the question Forsberg2016 as all such nuclides thus far produced exhibit decay chains which terminate in spontaneous fission prior to reaching well-known nuclides. Results from efforts to unambiguously determine using characteristic X-rays x-ray have yet to garner widespread acceptance, either. The Provisional Report of the 2017 Joint Working Group of IUPAC and IUPAP JWP2017 suggested that direct determination of the atomic mass with a sufficient precision could, in many cases, be a valid means to fully determine the and of an uncertain nuclide, particularly if decay information were simultaneously obtained. A first effort in this direction has recently shown some promise by directly verifying the mass number of a superheavy nuclide FIONA2018 , however without a level of mass precision needed to confirm the atomic number.
Beyond identification of SHN, the precise determination of atomic masses is vital to understanding the heaviest elements. Proper evaluation of the possible production – both in the laboratory Production_Sobiczewski ; Production_Oganessian and in the cosmos CosmicSHE_Petermann ; CosmicSHE_Flerov – of nuclides in the island of stability, theorized to be composed of exceptionally long-lived SHN Wheeler56 , requires accurate atomic masses in the heavy and superheavy region.
Among isotopes of transuranium elements, directly determined atomic masses are rare SHIPTRAP_No ; TRIGATRAP ; Ito2018 ; for SHN () directly determined atomic masses are completely absent. Atomic masses of most nuclides are determined indirectly, often through long decay chains. Herein we report the first direct measurement of the atomic mass of an SHN, 257Db. The measurement was performed by combination of a multi-reflection time-of-flight mass spectrograph (MRTOF-MS) and the newly developed “-TOF” detector Niwase2019 , greatly improving the sensitivity of the MRTOF-MS by providing correlational data between time-of-flight (ToF) and subsequent -decay. This measurement, the first to utilize -decay correlated time-of-flight mass spectroscopy, serves as both a cross-check for our previous indirect measurement of the mass of 257Db Ito2018 and a proof-of-principle for future efforts to measure nuclides in the hot-fusion superheavy island which do not connect to well-known nuclei via -decay, and where typical yields will be on the order of a few per day.
Atoms of 257Db were produced in the fusion-evaporation reaction 208Pb(51V, 2n)257Db Gates2008 . The RIKEN Ring Cyclotron provided a 306 MeV beam of 51V13+ with maximum intensity of 500 pnA. The beam impinged upon a rotating target wheel comprised of aluminum energy degraders and 208Pb targets. The targets were made from 208Pb enriched to 99.6% and deposited on a 30 g/cm2 carbon backing, with a typical lead thickness of 360 g/cm2. Aluminum energy degraders of 12 m thickness were utilized to reduce the beam energy to 243 MeV at target center. A detector angled 45∘ to the beam axis, located near the target wheel, measured the rate of elastic recoils from the target, providing a means to measure the effective primary beam dose.
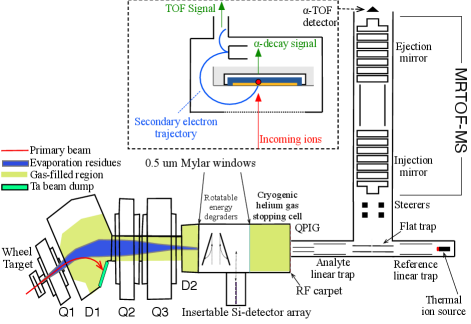
The gas-filled recoil ion separator GARIS-II GARIS-II transported 257Db while suppressing the primary beam and various transfer products. It was filled with dilute helium gas at 70 Pa. From previous experience with 257Db Brionnet257Db the selective dipole (D1 in Fig. 1) was set to 1.42 T.
As shown in Fig. 1, after exiting GARIS-II the beam passed through rotatable Mylar energy degraders prior to entering a helium-filled gas cell. The gas cell was cryogenically cooled to 60 K and pressurized to 200 mbar room temperature equivalent. The Mylar degrader thickness was chosen to reduce the energy of 257Db to be commensurate with the stopping power of the helium in the gas cell. A static electric field transported stopped ions to a traveling-wave radio-frequency (RF) ion carpet Wada2003 ; Arai2014 with a 0.74 mm diameter exit orifice. After exiting the gas cell, ions were transported through a differentially pumped region by use of quadrupole RF ion guides and trapped in a segmented linear Paul trap, which is part of a three-trap suite used to prepare analyte and reference ions for analysis by the MRTOF-MS using the concomitant referencing method Ito2018 ; SchuryWideband2 that allows analyte ions to be accumulated with nearly 100% duty cycle. The traps were cryogenically cooled to 150 K to minimize the probability of stored ions charge exchanging with residual background gases.
The MRTOF-MS, a device finding widespread use in recent years Knauer2019 ; Fischer2018 ; Wienholtz2013 ; Wolf2013a ; PLA2013457 ; Leistenschneider2018 ; HIRSH2016229 ; SCHULTZ2016251 ; CHAUVEAU2016 ; WANG2020179 ; Murray2019 , consists of a pair of ion mirrors separated by a field-free drift region. The outermost electrode of each mirror is switched to allow ions to enter and exit. Ions are stored in the MRTOF-MS for a time sufficient to allow the ions to reflect a specific number of times and achieve a time focus. During the measurement reported herein, the mass resolving power at the time focus was typically 250 000, with flight times of 10 ms for ions. To preclude detector dead time leading to undercounting that could affect the reference peak shape, the reference ion source was adjusted so as to detect one reference ion (85Rb+ or 133Cs+) per cycle on average.
Stable molecular ions produced in the gas cell or transfer products not removed by GARIS-II may have mass-to-charge ratios significantly differing from the analyte ion and will make fewer or more reflections than the analyte ions and may, by happenstance, appear at the same ToF as the analyte ions. As such, erroneous attribution is a concern with MRTOF-MS measurements SchuryWideband2 ; Fischer2018 . In the case of analyte ions detected at a rate of a few per day, however, confidence in the ability to exclude background noise (dark countsDCR , cosmic rays, and - or -decay from e.g. transfer product ions), or even extremely low-yield molecular ions with mass-to-charge ratio nearly identical to the analyte, becomes an issue of concern.
To overcome these issues we have developed a novel “-TOF” detector Niwase2019 based on a commercial MagneToF ion detector. Incoming ions strike a specially coated impact plate, which then releases secondary electrons. The secondary electrons are isochronously guided by a permanent magnet through an electron multiplier to produce a detectable ion impact signal. An ion’s time-of-flight, defined as the duration starting with ejection from the ion trap and ending with detection of the ion impact signal, is measured using a time-to-digital converter (MCS6 from FAST ComTec).
We have embedded a silicon PIN diode in the impact plate of a MagneToF ion detector. The PIN diode’s energy resolution is 140 keV. High-confidence measurements can be achieved by evaluating “-decay-correlated ToF events” in which an -decay event (“ single”) of a proper energy is observed within a proper duration subsequent to an ion impact signal (“ToF single”) with timing consistent with the expected analyte ion.
Since the -TOF detector’s location precludes -particle energy calibration by offline sources, 185Hg was produced via the 139La(51V, 5n) reaction prior to production of 257Db. The 5653 keV and 5372 keV -particles from the -decay of 185Hg 1976GrZC ; NNDC185Hg were used to calibrate the -TOF’s silicon PIN diode.
Separately, the incoming rate of 185Hg was measured on an insertable silicon PIN diode array located between GARIS-II and the gas cell. Using the measured rate of 185Hg2+ in MRTOF-MS time-of-flight spectra, the efficiency from gas cell through to -TOF was determined to be between 4% and 5% for ToF detection.
Ions impact the -TOF detector with an energy of 2 keV/, implanting a few angstroms deep and geometrically limiting the detection efficiency to 45%. The recoil from an -particle emitted toward the detector is sufficient to eject the atom from the detector surface. Thus sequential -particles along the decay chain cannot be observed. Fortunately, when an particle is emitted away from the detector, the daughter is generally not removed from the surface and there is a similar 45% probability for detection of the daughter’s -decay. The lifetimes of nuclides in the 257Db decay chain allow the evaluation to extend out four decays, through 245Es. Accounting for the -decay branching ratios of each nuclide Hessberger2001 ; Briselet2019 the total likelihood to detect one of the -decays in the 257Db decay chain would be 65%.
An initial effort to measure 257Db2+ (based on previous experience SchuryDoubleCharge ; Rosenbusch2018 ; Ito2018 ) produced no correlated event candidates after a dose on target of 4.71017 particles. As the National Institute of Standards and Technology (NIST) Atomic Spectra Database NIST lists the 3 ionization potential of Db as being 23.11.6 eV, compared to helium’s 24.6 eV 1st ionization potential, it was possible that 257Db3+ ions would be delivered from the gas cell. As such, the transport conditions were set for 85 and an effort was made to measure 257Db3+. During 105 hours of measurement, with a total dose of 1.11018 particles, a total of 14 decay-correlated event candidates were observed, which was consistent with the evaluated system efficiency.
A ToF gate such that ns, where is the expected ToF of 257Db3+ based on the 2016 Atomic Mass Evaluation (AME16) AME16 excludes superfluous ions. An energy gate of 7.0 MeV encompass all -decays from 245Es, 249Md, 253Lr, and 257Db (see top panel of Fig. 2). Any gated ToF single followed within 120 s by a gated -decay single was considered a correlated event candidate.
Figure 2 plots the correlated events observed in this work in terms of detected -decay energy and decay time; events are named in order of occurrence. The right panel of Fig. 2 shows the anticipated decay time probability distributions Schmidt1984 for each nuclide; multiple curves are shown for 257Db and 253Lr to represent known isomers. Similarly, the upper panel shows the detector response curves for each -decay which could be observed in the 257Db decay chain. The events form two clusters corresponding to 257Db or 253Lr and 249Md or 245Es.
The full spectrum (above 7 MeV) of singles is shown in grey in the top panel of Fig. 2. The large peak centered near 7.5 MeV is presumed to be from 211Po. While the =126 neutron shell closure in 208Pb may suppress direct production of 211gPo (=516 ms) at this beam energy, its decay parent 211At (=7.214 h) can be produced in the decay of extremely short-lived multi-nucleon transfer products 215Fr (=86 ns), 219Ac (=12 s), and 223Pa (=5.1 ms). While evidence of 211m,gPo2+ was identified in the time-of-flight spectra, its short-lived progenitors were not seen, likely having decayed before being extracted from the gas cell. Based on the 120 s coincidence window and 235 observed -decays commensurate with 211Po in the course of 105 hours of data accumulation, during which 37 ToF singles events in the vicinity of 257Db3+ were observed (see Fig. 3), we could expect to observe 3 coincidental correlations with 211Po decay. A similar evaluation NiwaseThesis indicates that less than one coincidental correlation would be expected for the higher-energy -decay signals. In consideration of this, we exclude events E4, E7, and E10 from our analysis of the atomic mass of 257Db as their energies and decay times are more consistent with 211Po than with any nuclide in the 257Db decay chain.
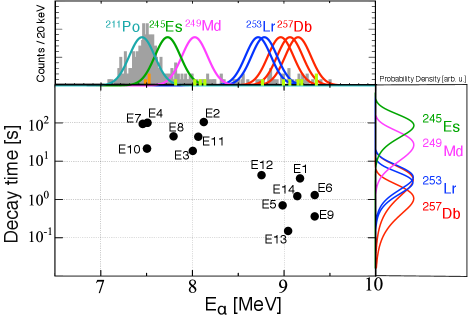
To determine the atomic mass, we typically make use of a single-reference method ItoSRM to evaluate the mass of an analyte ion using only one species of reference ion. The mass-to-charge ratio of the analyte ion can then be related to that of the reference ion by . The value is the actual experimental data, given by , where represents some inherent delay between the ions starting their movement in the analyzer and the start of the clock, while and are the times-of-flight of the analyte and reference, respectively. Based on ( 85Rb+ / 208Pb2+) measured at the end of the online experiment, it was determined that =75(4) ns.
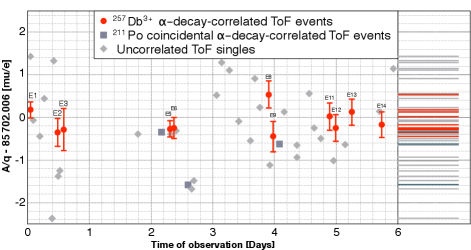
To exclude confusing an ion with significantly different for our intended analyte ion, spectra are typically made at different numbers of oscillations in the MRTOF-MS reflection chamber SchuryWideband2 . The times-of-flight and would typically be determined by fitting the analyte and reference ions’ spectra with a response function known to well-reproduce the data. In this work, however, it was not possible to perform such fittings on the analyte ions’ spectral peaks as the number of events at any given number of laps did not exceed three. Rather, for each analyte ion we made such a fitting for the reference ions spanning 7.5 s before and after the analyte ion’s detection and used the individual analyte ion’s ToF as . The result of this analysis is shown in Fig. 3, where the -decay-correlated ToF events are distinguished from uncorrelated events. The data for the -decay-correlated ToF events are tabulated in Table 1 along with the various characterizing qualities of each.
As the data is an admixture of spectra having differing flight paths, reliable fitting is precluded and we use algebraic weighted averaging to deduce of 257Db3+. The spectral peak is known to exhibit a slight asymmetry which could lead to a systematic error in such an evaluation. To ascertain the likely degree of such error, we used a data set comprising 3 358 consecutive sets of 10 analyte ions of 185Au2+ taken during preparation for the 257Db measurements. Mulltiplying the uncertainty of each data set by its Birge ratio Birge produced a nearly normal distribution: 51% were within 1- of the AME16-derived -value, 83% within 2-, 95% within 3-.
Event | laps | [MeV] | [s] | Nuclide | |
E1 | 300 | 9.19 | 3.54 | 257Db | 1.009 314 964(90) |
E2 | 300 | 8.14 | 105.00 | 249Md | 1.009 308 647(157) |
E3 | 300 | 8.02 | 18.50 | 249Md | 1.009 309 454(237) |
E5 | 325 | 9.00 | 0.70 | 257Db | 1.009 309 712(91) |
E6 | 325 | 9.35 | 1.30 | 257Db | 1.009 309 926(119) |
E8 | 324 | 7.81 | 44.00 | 245Es | 1.009 319 206(155) |
E9 | 324 | 9.35 | 0.36 | 257Db | 1.009 307 610(173) |
E11 | 327 | 8.08 | 43.40 | 249Md | 1.009 309 949(156) |
E12 | 327 | 8.77 | 4.30 | 253Lr | 1.009 313 092(150) |
E13 | 331 | 9.06 | 0.15 | 257Db | 1.009 314 345(148) |
E14 | 331 | 9.16 | 1.20 | 257Db | 1.009 310 844(144) |
Weighted Average | 1.009 311 901(40) | ||||
AME16-derived Value | 1.009 312 860(840) | ||||
Birge ratio | 24.4 | ||||
Reweighted Average | 1.009 311 901(973) |
After renormalizing the uncertainties, the weighted average ratio of was determined to be =1.009 311 901(973)(7), the systematic uncertainty deriving from =4 ns. This gives a mass excess of (2) keV/c2. The directly determined value differs from the previous value, which was determined indirectly from -values connecting 257Db to 249Md, by keV/c2. This indicates that the accepted -values for 257Db and 253Lr are accurate on the 100 keV level.
257Db exhibits at least one long-lived isomeric state Hessberger2001 . Neither the state order nor the isomeric excitation have been confirmed as yet. While NUBASE NUBASE2016 presently recommends an isomeric excitation of 140 keV based on systematics, -decay studies of 257Db populated by -decay of 261Bh suggest a 370 keV isomeric excitation Hessberger2010 . In this work, the mass resolution of our MRTOF nor the energy resolution of the -TOF detector were sufficient to resolve the two states in 257Db. As such, we must supplement the statistical uncertainty in the measured atomic mass with a systematic uncertainty accounting for the admixture of ground and isomer. A recent study of 257Db PierreThesis indicates an isomeric yield of 39(7)% for the shorter-lived state. As such, we assume the ToF-correlated -decay events measured were nearly evenly split between the two states. Splitting the difference between NUBASE and Ref. Hessberger2010 we therefore add 130 keV/c2 systematic uncertainty.
Among SHN, identification becomes ever more challenging with distance from species which could be produced in macroscopic quantities, particularly for production by hot fusion – where charge particle evaporation is more likely than in cold fusion – and in multinucleon transfer reactions. To demonstrate the degree to which the present technique may be applied to such a problem, consider Fig. 4. We present the span of =257 mass excess predictions from a comprehensive selection of global mass models DZ1 ; DZ ; FRDM12 ; WS4RBF ; HFB21 ; HFB32 ; WB03 ; KTUY05 (in blue), along with those from AME16 (in green). We superimpose on that the mass excess measured for each -decay-correlated ToF event presumed to correspond to 257Db3+.
In the 208Pb(51V, 2n)257Db reaction, the =257 nuclides which could be produced are limited by available neutrons and protons in the compound nucleus, as shown by the red box in Fig. 4. As such, it is clear that the analyte ion was 257Db. It is worth noting that, had a multi-nucleon transfer reaction been employed, the -TOF detector could use differences in the decay properties of 257Db and 257Am to distinguish between them.
Considering the demonstrated measurement precision and the typical variance of mass excess from theoretical models, it would be feasible to precisely determine in many – but certainly not all – cases of presently-known nuclides by -decay correlated ToF spectroscopy.
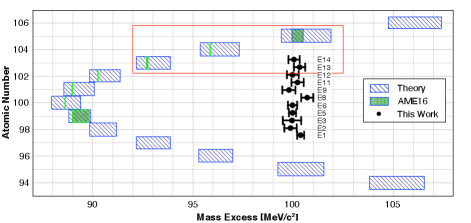
In this letter we have presented a new technique to mass analyze extremely low-yield species using -decay correlated ToF spectroscopy, and demonstrated a method of mass evaluation based on single-ions. Over the course of a five day online measurement we observed eleven 257Db correlated -ToF events, from which the mass excess of 257Db was determined to be (132) keV/c2 (=9.710-7), in good agreement with our previous indirect mass determination Ito2018 , and a direct determination of by comparison with mass models was demonstrated. Additionally, the observed predominance of triply-charged 257Db delivered from the helium gas cell indicates that the third ionization potential of dubnium must be less than 24.5 eV, restricting the range given by NIST NIST .
The techniques presented here will be used in future measurements to directly confirm the identities of hot-fusion superheavy nuclides sufficiently far from the valley of -decay stability, such as 288Mc/Fl, having sufficient separation to discern . Eventually, it may be applied to identification of multi-nucleon transfer products. Such reactions populate both sides of the valley of stability, making identification by mass spectroscopy impossible without utilizing decay correlated measurement.
To better resolve isomeric states in future measurements, efforts are underway to improve the energy resolution of the -TOF detector. Similarly, improvements in the MRTOF mass resolving power will allow the precision presented herein to be achieved with as few as 3 correlated -ToF events in future measurements; if the isomer in 257Db has an excitation energy exceeding 300 keV it could be resolved in the ToF spectrum.
We wish to express gratitude to the Nishina Center for Accelerator-Based Science at RIKEN and the Center for Nuclear Study at the University of Tokyo for their support of online measurements. This work was supported by the Japan Society for the Promotion of Science KAKENHI (Grant Numbers 2200823, 24224008, 24740142, 15H02096, 17H06090, 19K03899, 18H03711, and 15K05116).
References
- (1) Yu. Ts. Oganessian, F. Sh. Abdullin, S. N. Dmitriev, J. M. Gostic, J. H. Hamilton, R. A. Henderson, M. G. Itkis, K. J. Moody, A. N. Polyakov, A. V. Ramayya, J. B. Roberto, K. P. Rykaczewski, R. N. Sagaidak, D. A. Shaughnessy, I. V. Shirokovsky, M. A. Stoyer, N. J. Stoyer, V. G. Subbotin, A. M. Sukhov, Yu. S. Tsyganov, V. K. Utyonkov, A. A. Voinov, and G. K. Vostokin, Physical Review C 87 (2013) 014302
- (2) Yu. Ts. Oganessian, V. K. Utyonkov, Yu. V. Lobanov, F. Sh. Abdullin, A. N. Polyakov, I. V. Shirokovsky, Yu. S. Tsyganov,G. G. Gulbekian, S. L. Bogomolov, B. N. Gikal, A. N. Mezentsev, S. Iliev, V. G. Subbotin, A. M. Sukhov, A. A. Voinov,G. V. Buklanov, K. Subotic, V. I. Zagrebaev, and M. G. Itkis, J. B. Patin, K. J. Moody, J. F. Wild, M. A. Stoyer, N. J. Stoyer, D. A. Shaughnessy, J. M. Kenneally, and R. W. Lougheed, Physical Review C 69 (2004) 054607
- (3) Kosuke Morita, Kouji Morimoto, Daiya Kaji, Hiromitsu Haba, Kazutaka Ozeki, Yuki Kudou, Takayuki Sumita, Yasuo Wakabayashi, Akira Yoneda, Kengo Tanaka, Sayaka Yamaki, Ryutaro Sakai, Takahiro Akiyama, Shin-ichi Goto, Hiroo Hasebe, Minghui Huang, Tianheng Huang, Eiji Ideguchi, Yoshitaka Kasamatsu, Kenji Katori, Yoshiki Kariya, Hidetoshi Kikunaga, Hiroyuki Koura, Hisaaki Kudo, Akihiro Mashiko, Keita Mayama, Shin-ichi Mitsuoka, Toru Moriya, Masashi Murakami, Hirohumi Murayama, Saori Namai, Akira Ozawa, Nozomi Sato, Keisuke Sueki, Mirei Takeyama, Fuyuki Tokanai, Takayuki Yamaguchi, and Atsushi Yoshida, J. Phys. Soc. Jpn.81, 103201 (2012)
- (4) U. Forsberg, D. Rudolph, C. Fahlander, P. Golubev, L.G. Sarmiento, S. Åberg, M. Block, Ch.E. Düllmann, F.P. Heßberger, J.V. Kratz, A. Yakushev, Physics Letters B 760 (2016) 293-296.
- (5) D. Rudolph, U. Forsberg, P. Golubev, L. G. Sarmiento, A. Yakushev, L.-L. Andersson, A. Di Nitto, Ch. E. Düllmann, J. M. Gates, K. E. Gregorich, C. J. Gross, F. P. Heßberger, R.-D. Herzberg, J. Khuyagbaatar, J. V. Kratz, K. Rykaczewski, M. Schädel, S.Åberg, D. Ackermann, M. Block, H. Brand, B. G. Carlsson, D. Cox, X. Derkx, K. Eberhardt, J. Even, C. Fahlander, J. Gerl, E. Jäger, B. Kindler, J. Krier, I. Kojouharov, N. Kurz, B. Lommel, A. Mistry, C. Mokry, H. Nitsche, J. P. Omtvedt, P. Papadakis, I. Ragnarsson, J. Runke, H. Schaffner, B. Schausten, P. Thörle-Pospiech, T. Torres, T. Traut, N. Trautmann, A. Türler, A. Ward, D. E. Ward, and N. Wiehl, Physical Review Letters 111 (2013) 112502
- (6) Sigurd Hofmann, Sergey N. Dmitriev, Claes Fahlander, Jacklyn M. Gates, James B. Roberto and Hideyuki Sakai, Pure and Applied Chemistry 90 (2018) 1774–1832
- (7) J.M. Gates, G.K. Pang, J.L. Pore, K.E. Gregorich, J.T. Kwarsick, G. Savard, N.E. Esker, M. Kireeff Covo, M.J. Mogannam, J.C. Batchelder, D.L. Bleuel, R.M. Clark, H.L. Crawford, P. Fallon, K.K. Hubbard, A.M. Hurst, I.T. Kolaja, A.O. Macchiavelli, C. Morse, R. Orford, L. Phair, and M.A. Stoyer, Physical Review Letters 121 (2018) 222501
- (8) A. Sobiczewski and K. Pomorski, Prog. Part. Nucl. Phys 58 (2007) 292
- (9) Y. T. Oganessian, V. K. Utyonkov, Y. V. Lobanov, F. S. Abdullin, A. N. Polyakov, R. N. Sagaidak, I. V. Shirokovsky, Y. S. Tsyganov, A. A. Voinov, G. G. Gulbekian, S. L. Bogomolov, B. N. Gikal, A. N. Mezentsev, S. Iliev, V. G. Subbotin, A. M. Sukhov, K. Subotic, V. I. Zagrebaev, G. K. Vostokin, M. G. Itkis, K. J. Moody, J. B. Patin, D. A. Shaughnessy, M. A. Stoyer, N. J. Stoyer, P. A. Wilk, J. M. Kenneally, J. H. Landrum, J. F. Wild, and R. W. Lougheed, Physical Review C 74 (2006) 044602
- (10) I. Petermann, K. Langanke, G. Martínez-Pinedo, I. V. Panov, P.-G. Reinhard, F.-K. Thielemann, Eur. Phys. J. A 48 (2012) 122
- (11) G.N. Flerov, G.M. Ter-Akopian, Rep. Prog. Phys. 46 (1983) 817
- (12) J.A. Wheeler, Proc. Int. Conf. Peaceful Uses of Atomic Energy 2, 155, 220 (1956) (United Nations, New York)
- (13) Y. Ito, P. Schury, M. Wada, F. Arai, H. Haba, Y. Hirayama, S. Ishizawa, D. Kaji, S. Kimura, H. Koura, M. MacCormick, H. Miyatake, J.Y. Moon, K. Morimoto, K. Morita, M. Mukai, I. Murray, T. Niwase, K. Okada, A. Ozawa, M. Rosenbusch, A. Takamine, T. Tanaka, Y.X. Watanabe, H. Wollnik, and S. Yamaki, Physical Review Letters 120 (2018) 152501
- (14) M. Dworschak, M. Block, D. Ackermann, G. Audi, K. Blaum, C. Droese, S. Eliseev, T. Fleckenstein, E. Haettner, F. Herfurth, F. P. Heßberger1, S. Hofmann, J. Ketelaer, J. Ketter, H.-J. Kluge, G. Marx, M. Mazzocco, Yu. N. Novikov, W. R. Plaß, A. Popeko, S. Rahaman, D. Rodríguez, C. Scheidenberger, L. Schweikhard, P. G. Thirolf, G. K. Vorobyev, M. Wang, and C. Weber, Physical Review C 81 (2010) 064312
- (15) M. Eibach, T. Beyer, K. Blaum, M. Block, Ch. E. Düllmann,, K. Eberhardt, J. Grund, Sz. Nagy, H. Nitsche, W. Nörtershäuser, D. Renisch, K. P. Rykaczewski, F. Schneider, C. Smorra, J. Vieten, M. Wang, and K. Wendt, Physical Review C 89 (2014) 064318
- (16) T. Niwase, M. Wada, P. Schury, H. Haba, S. Ishizawa, Y. Ito, D. Kaji, S. Kimura, H. Miyatake, K. Morimoto, K. Morita, M. Rosenbusch, H. Wollnik, T. Shanley, Y. Benari, Nuclear Instruments and Methods in Physics Research, Section A: Accelerators, Spectrometers, Detectors and Associated Equipment, 953 (2019) 163198, DOI:10.1016/j.nima.2019.163198
- (17) J. M. Gates, S. L. Nelson, K. E. Gregorich, I. Dragojević, Ch. E. Düllmann, P. A. Ellison, C. M. Folden III, M. A. Garcia, L. Stavsetra, R. Sudowe, D. C. Hoffman, and H. Nitsche Phys. Rev. C 78 (2008) 034604
- (18) D. Kaji, K. Morimoto, N. Sato, A. Yoneda, K. Morita, Nuclear Instruments and Methods in Physics Research Section B: Beam Interactions with Materials and Atoms 317B (2013) 311-314
- (19) Private Communication with P. Brionnet
- (20) Michiharu Wada, Yoshihisa Ishida, Takashi Nakamura, Yasunori Yamazaki, Tadashi Kambara, Hitoshi Ohyama, Yasushi Kanai, Takao M. Kojima, Youichi Nakai, Nagayasu Ohshima, Atsushi Yoshida, Toshiyuki Kubo, Yukari Matsuo, Yoshimitsu Fukuyama, Kunihiro Okada, Tetsu Sonoda, Shunsuke Ohtani, Koji Noda, Hirokane Kawakami, and Ichiro Katayama, Nuclear Instruments and Methods in Physics Research Section B: Beam Interactions with Materials and Atoms 204 (2003) 570
- (21) F. Arai, Y. Ito, M. Wada, P. Schury, T. Sonoda, and H. Mita, Int. J. Mass Spectrom. 362 (2014) 56-58
- (22) P. Schury, Y. Ito, M. Rosenbusch, H. Miyatake, M. Wada, H. Wollnik, Int. J. Mass Spectrom. 433 (2018) 40-46
- (23) S. Knauer, P. Fischer, G. Marx, M. Müller, M. Rosenbusch, B. Schabinger, L. Schweikhard, R.N. Wolf, International Journal of Mass Spectrometry 446 (2019) 116189
- (24) Paul Fischer, Gerrit Marx, Lutz Schweickhard, International Journal of Mass Spectrometry 435 (2019) 305-314
- (25) Y. Kang, H. X. Lu, and Y.-H. Lo, Appl. Phys. Lett. 83, 2955 (2003)
- (26) F. Wienholtz, D. Beck, K. Blaum, Ch. Borgmann, M. Breitenfeldt, R.B. Cakirli, S. George, F. Herfurth, J.D. Holt, M. Kowalska, S. Kreim, D. Lunney, V. Manea, J. Menèndez, D. Neidherr, M. Rosenbusch, L. Schweikhard, A. Schwenk, J. Simonis, J. Stanja, R.N. Wolf, and K. Zuber, Nature 498 (2013) 346-349
- (27) R.N. Wolf, F. Wienholtz, D. Atanasov, D. Beck, K. Blaum, Ch. Borgmann, F. Herfurth, M. Kowalska, S. Kreim, Yu. A. Litvinov, D. Lunney, V. Manea, D. Neidherr, M. Rosenbusch, L. Schweikhard, J. Stanja, and K. Zube, International Journal of Mass Spectrometry 349-350 (2013) 349-350
- (28) W.R. Plaß, T. Dickel, S. Purushothaman, P. Dendooven, H. Geissel, J. Ebert, E. Haettner, C. Jesch, M. Ranjan, M.P. Reiter, H. Weick, F. Amjad, S. Ayet, M. Diwisch, A. Estrade, F. Farinon, F. Greiner, N. Kalantar-Nayestanaki, R. Knöbel, J. Kurcewicz, J. Lang, I. Moore, I. Mukha, C. Nociforo, M. Petrick, M. Pfützner, S. Pietri, A. Prochazka, A.-K. Rink, S. Rinta-Antila, D. Schäfer, C. Scheidenberger, M. Takechi, Y.K. Tanaka, J.S. Winfield, and M.I. Yavor, Nuclear Instruments and Methods in Physics Research Section B: Beam Interactions with Materials and Atoms 317 (2013) 457-462
- (29) E. Leistenschneider, M.P. Reiter, S. Ayet San Andrés, B. Kootte, J.D. Holt, P. Navrátil, C. Babcock, C. Barbieri, B.R. Barquest, J. Bergmann, J. Bollig, T. Brunner, E. Dunling, A. Finlay, H. Geissel, L. Graham, F. Greiner, H. Hergert, C. Hornung, C. Jesch, R. Klawitter, Y. Lan, D. Lascar, K.G. Leach, W. Lippert, J.E. McKay, S.F. Paul, A. Schwenk, D. Short, J. Simonis, V. Somà, R. Steinbrügge, S.R. Stroberg, R. Thompson, M.E. Wieser, C. Will, M. Yavor, C. Andreoiu, T. Dickel, I. Dillmann, G. Gwinner, W.R. Plaß, C. Scheidenberger, A.A. Kwiatkowski, J. and Dilling, Physical Review Letters 120 (2018) 062503
- (30) Tsviki Y. Hirsh, Nancy Paul, Mary Burkey, Ani Aprahamian, Fritz Buchinger, Shane Caldwell, Jason A. Clark, Anthony F. Levand, Lin Ling Ying, Scott T. Marley, Graeme E. Morgan, Andrew Nystrom, Rodney Orford, Adrian Pérez Galván, John Rohrer, Guy Savard and Kumar S. Sharma, Kevin Siegl, Nuclear Instruments and Methods in Physics Research Section B: Beam Interactions with Materials and Atoms 376 (2016) 229-232
- (31) B.E. Schultz, J.M. Kelly, C. Nicoloff, J. Long, S. Ryan, and M. Brodeur, Nuclear Instruments and Methods in Physics Research Section B: Beam Interactions with Materials and Atoms 376 (2016) 251-255
- (32) P. Chauveau, P. Delahaye, G. De France, S. El Abir, J. Lory, Y. Merrer, M. Rosenbusch, L. Schweikhard, and R.N. Wolf, Nuclear Instruments and Methods in Physics Research Section B: Beam Interactions with Materials and Atoms 376 (2016) 211-215
- (33) Jun-Ying Wang, Yu-Lin Tian, Yong-Sheng Wang, Zai-Guo Gan, Xiao-Hong Zhou, Hu-Shan Xu, and Wen-Xue Huang, Nuclear Instruments and Methods in Physics Research Section B: Beam Interactions with Materials and Atoms 463 (2020) 179-183
- (34) K. Murray, J. Dilling, R. Gornea, Y. Ito, T. Koffas, A.A. Kwiatkowski, Y. Lan, M.P. Reiter, V. Varentsov, T. Brunner, and the nEXO collaboration, Hyperfine Interactions 240 (2019) 97
- (35) J.W.Gruter, B.Jonson, O.B.Nielsen, the ISOLDE Collaboration - CERN-76-13, p.428 (1976)
- (36) S.-C. Wu, Nucl. Data Sheets 106 (2005) 619
- (37) R. Briselet, Ch. Theisen, M. Vandebrouck, A. Marchix, M. Airiau, K. Auranen, H. Badran, D. Boilley, T. Calverley, D. Cox, F. Déchery, F. Defranchi Bisso, A. Drouart, B. Gall, T. Goigoux, T. Grahn, P.T. Greenlees, K. Hauschild, A. Herzan, R.D. Herzberg, U. Jakobsson, R. Julin, S. Juutinen, J. Konki, M. Leino, A. Lightfoot, A. Lopez-Martens, A. Mistry, P. Nieminen, J. Pakarinen, P. Papadakis, J. Partanen, P. Peura, P. Rahkila, J. Rubert, P. Ruotsalainen, M. Sandzelius, J. Saren, C. Scholey, J. Sorri, S. Stolze, B. Sulignano, J. Uusitalo, A. Ward, and M. Zielińska, Physical Review C 99 (2019) 024614
- (38) F.P. Heßberger, S. Hofmann, D. Ackermann, V. Ninov, M. Leino, G. Münzenberg, S. Saro, A. Lavrentev, A.G. Popeko, A.V. Yeremin, and Ch. Stodel, The European Physical Journal A - Hadrons and Nuclei 12 (2001) 57-67
- (39) M. Rosenbusch, Y. Ito, P. Schury, M. Wada, D. Kaji, K. Morimoto, H. Haba, S. Kimura, H. Koura, M. MacCormick, H. Miyatake, J. Y. Moon, K. Morita, I. Murray, T. Niwase, A. Ozawa, M. Reponen, A. Takamine, T. Tanaka, and H. Wollnik, Physical Review C 97 (2018) 064306
- (40) P. Schury, M. Wada, Y. Ito, D. Kaji, H. Haba, Y. Hirayama, S. Kimura, H. Koura, M. MacCormick, H. Miyatake, J. Y. Moon, K. Morimoto, K. Morita, I. Murray, A. Ozawa, M. Rosenbusch, M. Reponen, A. Takamine, T. Tanaka, Y. X. Watanabe and H. Wollnik, Nuclear Instruments and Methods in Physics Research, Section B: Beam Interactions with Materials and Atoms, 407 (2017) 160-165, DOI:10.1016/j.nimb.2017.06.014
- (41) Kramida, A., Ralchenko, Yu., Reader, J., and NIST ASD Team (2019). NIST Atomic Spectra Database (ver. 5.7.1), [Online]. Available: https://physics.nist.gov/asd [2020, March 30]. National Institute of Standards and Technology, Gaithersburg, MD. DOI: https://doi.org/10.18434/T4W30F
- (42) M. Wang, G. Audi, F. G. Kondev, W. J. Huang, S. Naimi, and X. Xu, Chinese Physics C 41 (2017) 030003
- (43) K.-H. Schmidt, C.-C. Sahm, K. Pielenz, and H.-G. Clerc, Zeitschrift für Physik A 316 (1984) 19-26
- (44) Toshitaka Niwase, First direct mass measurement of superheavy nuclide via MRTOF mass spectrograph equipped with an -TOF detector, PhD. Thesis, Department of Physics, Kyushu University, February 2021
- (45) Y. Ito, P. Schury, M. Wada, S. Naimi, T. Sonoda, H. Mita, F. Arai, A. Takamine, K. Okada, A. Ozawa, and H. Wollnik, Physical Review C 88 (2013) 011306(R)
- (46) R.T. Birge, Phys. Rev. 40 (1932) 207
- (47) F.P. Heßberger, S. Hofmann, D. Ackermann, V. Ninov, M. Leino, G. Münzenberg, S. Saro, A. Lavrentev, A.G. Popeko, A.V. Yeremin, and Ch. Stodel, Eur. Phys. J. A (2001) 57-67
- (48) G. Audi, F. G. Kondev, Wang Meng, Huang W.J., and S. Naimi, Chinese Physics C 41 (2017) 030001
- (49) Pierre Brionnet, Etude des états isomères des noyaux superlourds :cas des noyaux 257Db et 253Lr, PhD. Thesis, Université de Strasbourg, September 2017
- (50) F. P. Heßberger, S. Antalic, D. Ackermann, S. Heinz, S. Hofmann, J. Khuyagbaatar, B. Kindler, I. Kojouharov, B. Lommel, and R. Mann, Eur. Phys. J. A 43 (2010) 175
- (51) J. Duflo and A. P. Zuker, Phys. Rev. C 52, R23 (1995)
- (52) J. Mendoza-Temis, J.G. Hirsch, A.P. Zuker, Nuc. Phys. A, Vol. 843, Issues 1-4, 2010, Pgs. 14-36
- (53) P. Möller, A. Sierk, T. Ichikawa, and H. Sagawa, At. Data Nucl. Data Tables 109 (2016) 1
- (54) N. Wang and M. Liu, Phys. Rev. C 84 (2011) 051303(R)
- (55) S. Goriely, N. Chamel, J. M. Pearson, Phys. Rev. C 82 (2010) 035804
- (56) S. Goriely, N. Chamel, and J. M. Pearson, Phys. Rev. C 93 (2016) 034337
- (57) H. Koura, T. Tachibana. Bulletin Physical Society of Japan (BUTSURI), 60 (2005) [Japanese language]
- (58) H. Koura, T.Tachibana, M. Uno, M. Yamada, Progr. Theor. Phys. 113 (2005) 305