Characterization of Sr2RuO4 Josephson junctions
made of epitaxial films
Abstract
We have studied fundamental properties of weak-link Sr2RuO4/Sr2RuO4 Josephson junctions fabricated by making a narrow constriction on superconducting Sr2RuO4 films through laser micro-patterning. The junctions show a typical overdamped behavior with much higher critical current density, compared with those previously reported for bulk Sr2RuO4/-wave superconductor junctions. Observed magnetic field and temperature dependences of the Josephson critical current suggest that the chiral -wave is unlikely for the superconducting symmetry, encouraging further theoretical calculations of the Sr2RuO4/Sr2RuO4 type junctions.
The discovery of superconductivity in Sr2RuO4 SRO has been followed by many important experimental and theoretical studies on unconventional superconductivity and its underlying physics, especially in terms of a possible chiral -wave order parameter symmetry SROreview1 ; SROreview3desiringfilm ; SROreview4desiringfilm . In the chiral -wave superconducting state, a finite orbital angular momentum of the paired electrons spontaneously breaks the time-reversal symmetry. The spin-triplet paring has been supported mainly by nuclear magnetic resonance (NMR) spectroscopy, observing unchanged Knight shift or spin susceptibility through the transition temperature SRO_NMR1 . The broken time-reversal symmetry has been indicated by muon spin relaxation (SR) SRO_usR and magneto-optical Kerr effect (MOKE) SRO_MOKE . On the other hand, there are other fundamental observations irreconcilable with the chiral -wave state. Suppression of the upper critical field, which may be caused by the paramagnetic pair breaking for the in-plane magnetic field, is a typical example Hc2_yonezawafirst1 ; Hc2_thinfilm ; SROreview4desiringfilm . In addition, chiral currents, emerging on the edge of chiral domains, have not been directly detected even by scanning superconducting quantum interference device (SQUID) microscopy with ultrahigh sensitivity SRO_nochiralcurrent1 ; SRO_nochiralcurrent2 . While this result could be explained by mixed chiral domains with small sizes ( m), the assumed domain sizes are substantially different even between the SR ( m) and MOKE ( m) experiments TRSB . Recent thermal conductivity and specific heat measurements also favor other -wave order parameters with line nodes SRO_specificheat ; SRO_thermalconductivity . Furthermore, a pronounced drop of the in-plane spin susceptibility has been recently confirmed by lower excitation power NMR experiments, which particularly rules out the possibility of the chiral -wave state SRO_NMR2 . In this context, development of Sr2RuO4 film-based junctions has been increasingly demanded for examining its superconducting state SROreview3desiringfilm ; SROreview4desiringfilm .
Phase sensitive experiments are capable of providing clear evidences for elucidating the superconducting pairing symmetry textbook1 . In the case of high- cuprates, for example, Josephson junctions fabricated from their epitaxial films have lead the determination of -wave order parameter symmetry. On the other hand, successful growth of superconducting Sr2RuO4 films was highly limited since its discovery YoshiharuPLD ; RobinsonPLD , and thus only bulk-based Josephson junctions, consisting of a Sr2RuO4 bulk processed by focus ion beam (FIB) milling and a -wave superconductor such as Pb SROJ_jin ; SROJ_kidwingira ; SROJ_nakagawa , Sn SROJ_sumiyama , and Nb SROJ_sumiyama ; SROJ_nago ; SROJ_saitoh1 ; SROJ_saitoh2 ; SROJ_anwar ; SROJ_kashiwaya_arxiv have long been studied. Such junctions have very large junction areas, compared to conventional film-based junctions. They are not a Sr2RuO4/Sr2RuO4 Josephson junction, which facilitates simple analysis in theoretical models SROJ_p-p1 ; SROJ_p-p2 ; SROJ_p-p3 ; SROJ_p-p4 without considering possible mutual proximity effects in the Sr2RuO4/-wave-superconductor junctions SROJ_s-p1 ; SROJ_s-p2 ; SROJ_s-p3 ; SROJ_s-p4 . It has also been reported that the the FIB process often damages the Sr2RuO4 bulks, particularly forming other superconducting phase called the 3-K phase. However, recent development of molecular beam epitaxy growth of high-quality superconducting Sr2RuO4 thin films UchidaMBE ; TopoOxidereview ; HariMBE , as well as characterization of fundamental superconducting properties of the films Hc2_thinfilm , is opening up a new route to study the unconventional superconductivity through film-based junction experiments.
Here we report fundamental properties of weak-link Sr2RuO4/Sr2RuO4 Josephson junctions, fabricated from high-quality Sr2RuO4 films with laser micro-patterning. Planar tunnel junctions are other prototypical example, but which is rather difficult to make, because it needs epitaxial growth of high-quality Sr2RuO4 films on an appropriate pin-hole free insulating barrier layer. The present film-based weak-link junctions, excluding possible proximity effects in the previous bulk-based junctions, are expected to enable more straight interpretation of characterization results.
Superconducting single crystalline Sr2RuO4 films with -axis orientation were grown by molecular beam epitaxy with a thickness of nm on (001) (LaAlO3)0.3(SrAl0.5Ta0.5O3)0.7 (LSAT) substrates in a Veeco GEN10 system UchidaMBE . 4N Sr and 3N5 Ru elemental fluxes were simultaneously supplied from a conventional Knudsen cell and a Telemark TT-6 electron beam evaporator, respectively. Molecular flux was calibrated using an INFICON quartz crystal microbalance system. The growth was performed at a substrate temperature of 900 ∘C, regulated with a semiconductor-laser heating system, and with flowing pure with a pressure of Torr, supplied from a Meidensha Co. MPOG-104A1-R ozone generator. Figure 1(a) shows x-ray diffraction (XRD) -2 scan of a Sr2RuO4 film used the junction fabrication. It shows only sharp (00) Sr2RuO4 peaks (: even integer) up to (0014) and no discernible impurity peaks due to slightly excess supply of Ru. This indicates that single-crystalline and stoichiometric Sr2RuO4 is almost homogeneously grown over the wide film area. Junctions with a narrow constriction were fabricated by selectively ablating a part of Sr2RuO4 films, with the irradiation of a second harmonic Nd:YAG laser (532 nm wave length, 5 nsec duration) for 10 seconds at a repetition frequency of 30 Hz. The pulsed laser beam was focused down to 1 m spot through optical slits and collective lenses and scanned at 250 nm steps to make the constriction with a width of m. Aluminum wires for the four-terminal measurement were connected using a wire bonding machine. The junctions were cooled down to 60 mK in an Oxford Instruments Kelvinox MX100 3He-4He dilution refrigerator equipped with a superconducting magnet. Voltage across the constriction was measured with a Keithley 2182A nanovoltmeter while flowing a DC current along the -axis with a Yokogawa 7651 programmable source.
Figure 1(b) shows temperature dependence of the in-plane resistivity of the Sr2RuO4 film used for the junction fabrication. It exhibits a superconducting transition at a midpoint critical temperature of K with a width of K. These values are the best ones among reported unstrained films grown on the cubic LSAT substrate YoshiharuPLD ; RobinsonPLD ; UchidaMBE ; Hc2_thinfilm . The epitaxial strain from the LSAT substrate is almost biaxial and a change in the in-plane lattice parameters compared to bulk is further suppressed at low temperatures ( %) Hc2_thinfilm , not requiring the consideration of uniaxial strain effect HariMBE ; Hc2strain in the present experiment. Figure 1(c) displays a Sr2RuO4/Sr2RuO4 junction fabricated from this film, where two Sr2RuO4 superconductor banks are connected through a narrow constriction. As confirmed in Fig. 1(d), the superconducting transition in the junction starts only slightly below the onset critical temperature of the original film. The laser fabrication process successfully avoids serious damage on the film, particularly formation of the 3-K phase as caused by the FIB process.
Figure 1(e) summarizes - characteristics and its temperature dependence observed in the Sr2RuO4/Sr2RuO4 junction supplement . It shows a typical overdamped behavior of Josephson junctions, with A and a normal state resistance of m at the base temperature. product, expressed by the product of and as a measure of the superconducting coupling strength, is V, which is comparable to the values previously reported for bulk-based Sr2RuO4 junctions SROJ_saitoh1 ; SROJ_saitoh2 ; SROJ_kidwingira ; SROJ_sumiyama ; SROJ_kashiwaya_arxiv but still lower than the reported superconducting gap amplitude of about – eV SRO_STMgap1 ; SRO_STMgap2 . On the other hand, the junction cross-sectional area in the present study is largely reduced to m2, typically by two to four orders of magnitude compared to the previously reported bulk-based junctions SROJ_saitoh1 ; SROJ_saitoh2 ; SROJ_kidwingira ; SROJ_anwar ; SROJ_kamabara1 ; SROJ_nago ; SROJ_kashiwaya_arxiv . Namely, much lower normalized resistance of about cm2 and higher critical current density of about A/cm2 are achieved in the present Josephson junction, probably because of the all-Sr2RuO4 structure avoiding interface problems.
As shown in Fig. 2(a), shows clear modulations responding to the applied magnetic field. In thin films of type II superconductors, a characteristic length scale of the magnetic field distribution around a vortex is given not by the London penetration depth , but by the Pearl penetration depth especially in the thin limit () textbook2 . Considering that in Sr2RuO4 bulks is about nm at the base temperature SRO_pd1_sans ; SRO_pd2_usR ; SRO_pd3_msi , modulation period in the conventional Fraunhofer pattern is estimated between Oe and Oe for the present junction. The fine oscillations appearing at intervals of about 15 Oe as seen in Figs. 2(b) and (c) are ascribed to this type of modulation. The oscillation pattern has been reproduced after wide sweeping of the magnetic field or recooling through the transition temperature, excluding possible domain contributions appearing as hysteresis in the bulk-based large junctions SROJ_kidwingira ; SROJ_saitoh2 ; SROJ_kamabara1 .
Figure 3(a) plots magnetic field dependence of , extracted from the map in Fig. 2(a). Here we conduct symmetrical analysis for the obtained curves by two types of operation. One is time-reversal operation, where both the current and field directions are inverted for taken at negative bias current, and then compared to taken at positive one (Fig. 3(b)). The other is current-reversal operation, where only the current direction is inverted for and then compared to (Fig. 3(c)). As confirmed in the comparison between and in Fig. 3(b), the observed oscillation patterns are almost unchanged by the time-reversal operation. This is in contrast to the case of the current-reversal operation in Fig. 3(c), where such a fine agreement of the oscillation patterns is not discerned between and . This mismatch is mainly ascribed to extrinsic self-field effect, where the trapped vortices impose a nonuniform contribution to the magnetic field. The oscillation curves and are then shifted in the opposite field direction, while time-reversal symmetry of the oscillation pattern is still preserved. In the case of chiral--wave/-wave corner and planar Josephson junctions without time-reversal symmetry, time-reversal symmetry breaking oscillation patterns have been theoretically expected from a multiband model calculation including the spin-orbit interaction SROJ_s-p4 ; SROJ_kashiwaya_arxiv . These patterns have been ascribed to symmetrically allowed cosine terms in the current-phase relation. However, the observed oscillation pattern is time-reversal invariant, and which cannot be explained by the mixed chiral domains, because such domain sizes become comparable to or even smaller than the in-plane coherence length SROreview1 ; Hc2_thinfilm . On the other hand, the time-reversal invariant oscillations do not directly indicate that time-reversal symmetry is preserved in the Sr2RuO4 system. In particular, so far it is not trivial that the above calculation based on the anomalous current-phase relation is simply applicable to other combinations of time-reversal symmetry breaking superconducting states such as chiral -wave.
Finally, we discuss temperature dependence of the product. For weak-link junctions, the Kulik-Omelyanchuk model based on a point contact has been proposed, and temperature dependence of the product has been theoretically derived for combination of -wave superconductors IcRn_KO5 . On the other hand, the ideal magnitude relation between the in-plane coherence length and junction width () is not satisfied in the present junction. As shown in Fig. 4, the observed temperature dependence seems deviated from the Kulik-Omelyanchuk relation in the present diffusive limit () for the mean-free path of about 50 nm Hc2_thinfilm . Tunnel-type junctions have been addressed by the Ambegaokar-Baratoff model, based on tunneling through a sandwiched insulator, but the observed temperature dependence is also further deviated from the relation derived for -wave combination. In chiral -wave tunnel junctions, on the other hand, it has been theoretically predicted that increases rapidly with approaching the base temperature, particularly for combination of the same chirality SROJ_p-p2 . However, such increase at low temperatures is not observed in the Sr2RuO4/Sr2RuO4 Josephson junction. Although detailed temperature dependence may be dependent on multiband or anisotropic effects, the chiral -wave state is unlikely also in terms of the temperature dependence.
In summary, we have fabricated the weak-link Sr2RuO4/Sr2RuO4 Josephson junctions with enhanced critical current density, and investigated the fundamental properties including the responses to magnetic field and temperature changes. Observed magnetic field and temperature dependences in the present Sr2RuO4/Sr2RuO4 Josephson junctions suggest that the chiral -wave is unlikely for the superconducting symmetry, while general time-reversal symmetry breaking states cannot be ruled out. The results obtained on the first all-Sr2RuO4 junction encourage further theoretical calculations considering other possible superconducting symmetry candidates SRO_NMR2 ; otherlist . The potential of the film-based all-Sr2RuO4 junctions has been also successfully demonstrated for further advancing studies by development of SQUID SROJ_s-p3 ; SROJ_s-p4 and grain boundary Josephson junctions J_p-FM1 ; J_p-FM3 , as well as theoretical analyses of the Sr2RuO4/Sr2RuO4 junction properties.
We acknowledge fruitful discussions with N. Nagaosa, R. Arita, J. Akimitsu, E. Zeldov, D. Manske, S. Ikegaya, K. Kanoda, Y. Iwasa, T. Shibauchi, T. Nojima, and S. Kittaka. This work was supported by Grant-in-Aids for Scientific Research (B) No. JP18H01866 and Scientific Research on Innovative Areas “Topological Materials Science” No. JP16H00980 from MEXT, Japan and JST PRESTO No. JPMJPR18L2 and CREST Grant No. JPMJCR16F1, Japan.
References
- (1) Y. Maeno, H. Hashimoto, K. Yoshida, S. Nishizaki, T. Fujita, J. G. Bednorz, and F. Lichtenberg, Nature 372, 532 (1994).
- (2) A. P. Mackenzie and Y. Maeno, Rev. Mod. Phys. 75, 657–712 (2003).
- (3) Y. Liu and Z. Q. Mao, Physica C 514, 339–353 (2015).
- (4) A. P. Mackenzie, T. Scaffidi, C. W. Hicks, and Y. Maeno, npj Quantum Mater. 2, 40-1–9 (2017).
- (5) K. Ishida, H. Mukuda, Y. Kitaoka, K. Asayama, Z. Q. Mao, Y. Mori, and Y. Maeno, Nature 396, 658–660 (1998).
- (6) G. M. Luke, Y. Fudamoto, K. M. Kojima, M. I. Larkin, J. Merrin, B. Nachumi, Y. J. Uemura, Y. Maeno, Z. Q. Mao, Y. Mori, H. Nakamura, and M. Sigrist, Nature 394, 558–561 (1998).
- (7) J. Xia, Y. Maeno, P. T. Beyersdorf, M. M. Fejer, and A. Kapitulnik, Phys. Rev. Lett. 97, 167002 (2006).
- (8) S. Yonezawa, T. Kajikawa, and Y. Maeno, Phys. Rev. Lett. 110, 077003 (2013).
- (9) M. Uchida, M. Ide, M. Kawamura, K. S. Takahashi, Y. Kozuka, Y. Tokura, and M. Kawasaki, Phys. Rev. B 99, 161111(R) (2019).
- (10) J. R. Kirtley, C. Kallin, C. W. Hicks, E.-A. Kim, Y. Liu, K. A. Moler, Y. Maeno, and K. D. Nelson, Phys. Rev. B 76, 014526 (2007).
- (11) C. W. Hicks, J. R. Kirtley, T. M. Lippman, N. C. Koshnick, M. E. Huber, Y. Maeno, W. M. Yuhasz, M. Brian Maple, and K. A. Moler, Phys. Rev. B 81, 214501 (2010).
- (12) C. Kallin and A, J. Berlinsky, J. Phys.: Condens. Matter 21, 164210 (2009).
- (13) S. Kittaka, S. Nakamura, T. Sakakibara, N. Kikugawa, T. Terashima, S. Uji, D. A. Sokolov, A. P. Mackenzie, K. Irie, Y. Tsutsumi, K. Suzuki, and K. Machida, J. Phys. Soc. Jpn. 87, 093703 (2018).
- (14) E. Hassinger, P. Bourgeois-Hope, H. Taniguchi, S. René de Cotret, G. Grissonnanche, M. S. Anwar, Y. Maeno, N. Doiron-Leyraud, and L. Taillefer, Phys. Rev. X 7, 011032 (2017).
- (15) A. Pustogow, Y. Luo, A. Chronister, Y.-S. Su, D. Sokolov, F. Jerzembeck, A. P. Mackenzie, C. W. Hicks, N. Kikugawa, S. Raghu, E. D. Bauer, and S. E. Brown, Nature doi: 10.1038/s41586-019-1596-2 (2019).
- (16) K. H. Bennemann and J. B. Ketterson, The Physics of Superconductors (Springer, Berlin, 2003).
- (17) Y. Krockenberger, M. Uchida, K. S. Takahashi, M. Nakamura, M. Kawasaki, and Y. Tokura, Appl. Phys. Lett. 97, 082502 (2010).
- (18) J. Cao, D. Massarotti, M. E. Vickers, A. Kursumovic, A. Di Bernardo, J. W. A. Robinson, F. Tafuri, J. L. MacManus-Driscoll, and M. G. Blamire, Supercond. Sci. Technol. 29, 095005 (2016).
- (19) R. Jin, Y. Zadorozhny, Y. Liu, D. G. Schlom, Y. Mori, and Y. Maeno, Phys. Rev. B 59, 4433 (1999).
- (20) F. Kidwingira, J. D. Strand, D. J. Van Harlingen, and Y. Maeno, Science 314, 1267–1271 (2006).
- (21) R. Nakagawa, T. Nakamura, T. Terashima, S. Yonezawa, and Y. Maeno, Physica C 470, S744–S745 (2010).
- (22) A. Sumiyama, T. Endo, Y. Oda, Y. Yoshida, A. Mukai, A. Ono,Y. Onuki, Physica C 367, 129–132 (2002).
- (23) Y. Nago, R. Ishiguro, T. Sakurai, M. Yakabe, T. Nakamura, S. Yonezawa, S. Kashiwaya, H. Takayanagi, and Y. Maeno, Phys. Rev. B 94, 054501 (2016).
- (24) K. Saitoh, S. Kashiwaya, H. Kashiwaya, M. Koyanagi, Y. Mawatari, Y. Tanaka, and Y. Maeno, Appl. Phys. Express 5, 113101 (2012).
- (25) K. Saitoh, S. Kashiwaya, H. Kashiwaya, Y. Mawatari, Y. Asano, Y. Tanaka, and Y. Maeno, Phys. Rev. B 92, 100504(R) (2015).
- (26) M. S. Anwar, T. Nakamura, S. Yonezawa, M. Yakabe, R. Ishiguro, H. Takayanagi, and Y. Maeno, Sci. Rep. 3, 2480 (2013).
- (27) S. Kashiwaya, K. Saitoh, H. Kashiwaya, M. Koyanagi, M. Sato, K. Yada, Y. Tanaka, and Y. Maeno, Phys. Rev. B 100, 094530 (2019).
- (28) Y. S. Barash, A. M. Bobkov, and M. Fogelström, Phys. Rev. B 64, 214503 (2001).
- (29) Y. Asano and K. J. Katabuchi, Phys. Soc. Jpn. 71, 1974–1977 (2002).
- (30) T. Yokoyama, Y. Tanaka, and A. A. Golubov, Phys. Rev. B 75, 094514 (2007).
- (31) Y. Sawa, T. Yokoyama, Y. Tanaka, and A. A. Golubov, Phys. Rev. B 75, 134508 (2007).
- (32) Y. Hasegawa, J. Phys. Soc. Jpn. 67, 3699–3702 (1998).
- (33) Y. Asano, Y. Tanaka, M. Sigrist, and S. Kashiwaya, Phys. Rev. B 67, 184505 (2003).
- (34) Y. Asano, Y. Tanaka, M. Sigrist, and S. Kashiwaya, Phys. Rev. B 71, 214501 (2005).
- (35) K. Kawai, K. Yada, Y. Tanaka, Y. Asano, A. A. Golubov, and S. Kashiwaya, Phys. Rev. B 95, 174518 (2017).
- (36) M. Uchida, M. Ide, H. Watanabe, K. S. Takahashi, Y. Tokura, and M. Kawasaki, APL Mater. 5, 106108-1–5 (2017).
- (37) M. Uchida and M. Kawasaki, J. Phys. D: Appl. Phys. 51, 143001 (2018).
- (38) H. P. Nair, J. P. Ruf, N. J. Schreiber, L. Miao, M. L. Grandon, D. J. Baek, B. H. Goodge, J. P. C. Ruff, L. F. Kourkoutis, K. M. Shen, and D. G. Schlom, APL Mater. 6, 101108 (2018).
- (39) A. Steppke, L. Zhao, M. E. Barber, T. Scaffidi, F. Jerzembeck, H. Rosner, A. S. Gibbs, Y. Maeno, S. H. Simon, A. P. Mackenzie, and C. W. Hicks, Science 355, eaaf9398 (2017).
- (40) See Supplemental Material at [URL will be inserted by publisher] for additional current-voltage sweeps.
- (41) M. D. Upward, L. P. Kouwenhoven, A. F. Morpurgo, N. Kikugawa, Z. Q. Mao, and Y. Maeno, Phys. Rev. B 65, 220512(R) (2002).
- (42) H. Suderow, V. Crespo, I. Guillamon, S. Vieira, F. Servant, P. Lejay, J. P. Brison, and J. Flouquet, New J. Phys. 11, 093004 (2009).
- (43) H. Kambara, S. Kashiwaya, H. Yaguchi, Y. Asano, Y. Tanaka, and Y. Maeno, Phys. Rev. Lett. 101, 267003 (2008).
- (44) M. Tinkham, Introduction to Superconductivity (Dover, New York, 2004).
- (45) T. M. Riseman, P. G. Kealey, E. M. Forgan, A. P. Mackenzie, L. M. Galvin, A. W. Tyler, S. L. Lee, C. Ager, D. McK. Paul, C. M. Aegerter, R. Cubitt, Z. Q. Mao, T. Akima, and Y. Maeno, Nature 396, 242–245 (1998).
- (46) G. M. Luke, Y. Fudamoto, K. M. Kojima, M. I. Larkin, B. Nachumi, Y. J. Uemura, J. E. Sonier, Y. Maeno, Z.Q. Mao, Y. Mori, and D. F. Agterberg, Physica B 289–290 373–376 (2000).
- (47) P. J. Baker, R. J. Ormeno, C. E. Gough, Z. Q. Mao, S. Nishizaki, and Y. Maeno, Phys. Rev. B 80, 115126 (2009).
- (48) A. A. Golubov, M. Y. Kupriyanov, and E. Il’ichev, Rev. Mod. Phys. 76, (2004).
- (49) H. S. Røising, T. Scaffidi, F. Flicker, G. F. Lange, and S. H. Simon, arXiv:1907.09485 (2019).
- (50) Y. Asano, Phys. Rev. B 74, 220501(R) (2006).
- (51) D. Manske and P. M. R. Brydon, J. Phys.: Conf. Ser. 108, 012042 (2008).
- (52) V. Ambegaokar and A. Baratoff, Phys. Rev. Lett. 10, 486 (1963).
- (53) S. Shirai, H. Tsuchiura, Y. Asano, Y. Tanaka, J. Inoue, Y. Tanuma, and S. Kashiwaya, J. Phys. Soc. Jpn. 72, 2299–2307 (2003).
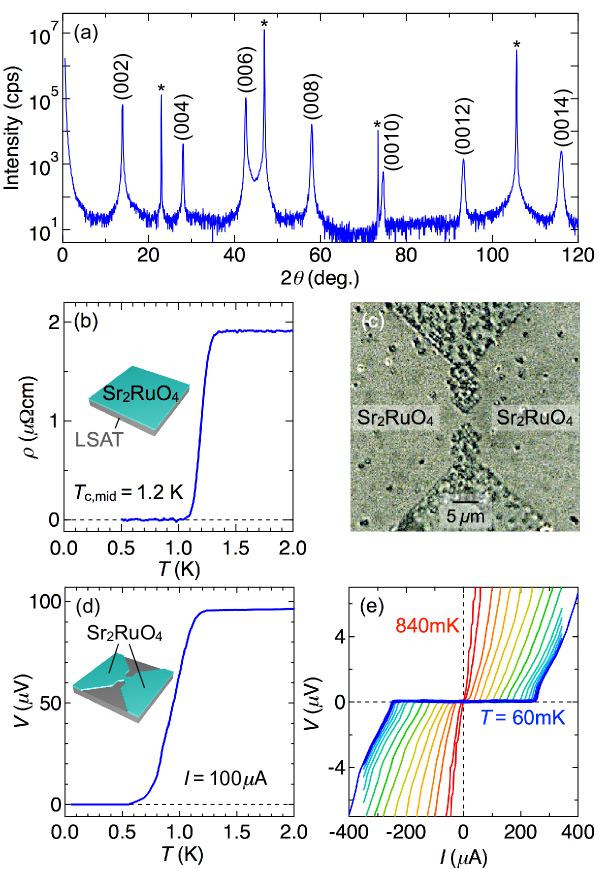
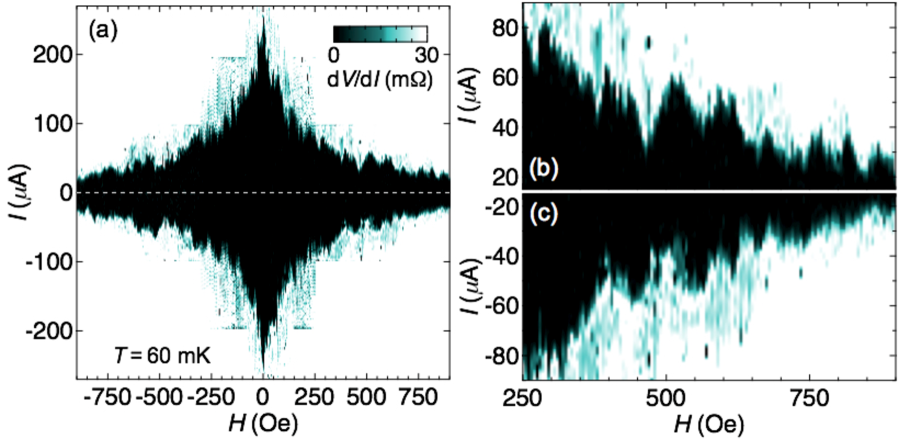
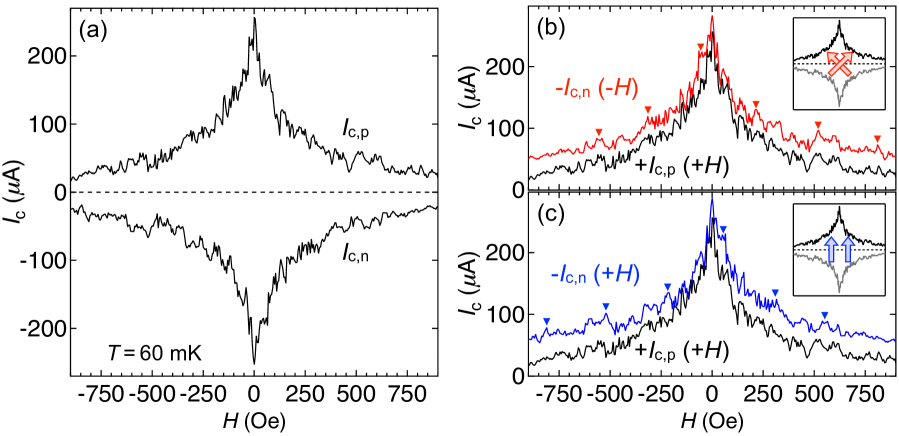
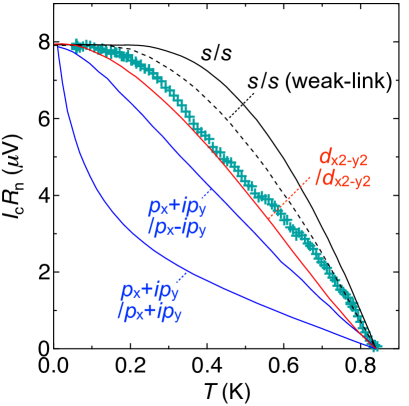