A scheme to create and verify scalable entanglement in optical lattice
I Abstract
To achieve scalable quantum information processing, great efforts have been devoted to the creation of large-scale entangled states in various physical systems. Ultracold atom in optical lattice is considered as one of the promising platforms due to its feasible initialization and parallel manipulation. In this work, we propose an efficient scheme to generate and characterize global entanglement in the optical lattice. With only two-layer quantum circuits, the generation utilizes two-qubit entangling gates based on the superexchange interaction in double wells. The parallelism of these operations enables the generation to be fast and scalable. To verify the entanglement of this non-stabilizer state, we mainly design three complementary detection protocols which are less resource-consuming compared to the full tomography. In particular, one just needs two homogenous local measurement settings to identify the entanglement property. Our entanglement generation and verification protocols provide the foundation for the further quantum information processing in optical lattice.
II Introduction
Quantum information and quantum computation [1], which harvest the intrinsic quantum features, like superposition and entanglement [2], can show advantages against their classical counterparts. To build a practical quantum information processor, the computation platform with high scalability is preferred and the qubits should be coupled to each other to form a large-scale entanglement. Hence, many researches have been focusing on the generation of scalable entangled states in different physical systems, e.g, ion trap [3, 4, 5], photons [6, 7], Rydberg atoms [8] and superconducting circuits [9, 10, 11]. Even though there are significant progresses of the qubit number in various systems [12, 13], the generation of large-scale entanglement is still challenging for the Noisy Intermediate Scale Quantum Devices [14].
Ultracold atoms in optical lattice [15] could be a practicable system to overcome this challenge due to its feasible initialization and parallel manipulation. By adiabatically increasing the lattice depth, the phase of ultracold atom can be tuned from superfluid (SF) to Mott insulator (MI) [16, 17]. Under an unit filling rate, numerous atoms can be confined in the lattice and serves as qubits. Based on this initialization, entangled states in optical lattice have been demonstrated experimentally, for instance, the generation of cluster state with controlled collision gate induced by the spin-dependent lattice [18, 19], which acts as the resource state for the measurement-based quantum computing [20, 21]. The development of superlattice further improves the ability to control ultracold atoms, which is formed by overlapping two different optical lattices to generate a series of double wells. The structure of the double well can be modified to induce different kinds of atomic dynamics, such as superexchange coupling [22] and controlled exchange interaction [23], which can be both used to realize gate and entangle two atoms in a double well [24, 23]. Besides, the entangling operations in the superlattice can be performed in a parallel way based on the periodicity of the lattice system, which is suitable for the fast generation of large amount of entangled pairs and even large-scale entangled states. Note that this kind of parallel operation can also be implemented with multi-tweezer in Rydberg atom experiments [25, 26].
Every coin has two sides. The periodicity property also induces some restrictions on the quantum operation and measurement. The small lattice spacing, required by the large tunnelling between neighbour sites, creates a challenge for the individual control of atoms, say, some local basis rotations. The tight-focused optical tweezer [27] created with the recently developed high-resolution imaging [28, 29, 30, 31, 32] could be a solution. However, it is still challenging to perform a few of different single-qubit operations on multiple qubits under a realistic time-scale to ensure the system coherence. As a result, homogeneous operations and measurements on all atoms are preferred in optical lattice experiments.
In this work, we propose a scheme to generate scalable entanglement of ultracold atoms which is suitable for the implementation in the optical superlattice system. It mainly contains two entangling steps: first, entangle the atom-pair in each double well by the gate; second, shift the position of double wells to a single site by changing the phase of superlattice, and then entangle the the new atom-pair with again. In this way, all atoms in the superlattice can be connected with neighbours to form a global entangled state. Our theoretical analysis shows that the final state possesses genuine multipartite entanglement (GME). In addition, the final state is also less sensitive to magnetic noises which can cause decoherence, since it only owns amplitude on the computational basis whose total spin is zero.
In actual experiments, the inevitable noise may degrade the entanglement, which should be verified further. Compared with quantum tomography [33, 34], entanglement witness is a more efficient way [35, 36, 37] to realize this task based on the pre-knowledge of the preparation. Current entanglement witnesses are usually designed for some structured states, such as permutation-invariant states [38, 39, 40] and stabilizer states [41, 42, 43]. However, in our protocol the state generated by the non-Clifford gate is not a standard stabilizer state, which makes the entanglement verification challenging. To overcome this challenge, we first construct an entanglement witness based on the preparation fidelity. By adopting the decomposition using stabilizer-like method, we can lower bound the fidelity with a few spin-correlation measurements. Some correlations among them are inhomogenous and thus require the individual atom addressing whose implementation could be challenging with current techniques. To further ease the experiment realization, we show another complementary protocol which only requires homogenous spin-correlations and only two measurement settings. At last, by reversely evolving the state with the conjugate quantum gates, we provide an intuitive method to indirectly bound the preparation fidelity and thus qualitatively verify the entanglement.
III Results
III.1 Entangling Gates in Optical Superlattices
The behavior of ultracold atoms in optical lattices can be described by the Hubbard model which is characterized by the tunnel coupling between neighbouring sites , the on-site interaction , and the effective chemical potential . By increasing the depth of the lattice, a phase transition from SF to MI can occur and the atoms start to be localized in each site. To extend the ability to manipulate atoms, a more complicated periodic potential, named superlattice, was proposed and applied in many experiments [44, 45, 46, 47]. It is normally formed by overlapping two distinct optical lattices. The period of the first lattice (denoted as the long lattice) is twice as that of the second (denoted as the short lattice), which induces an array of double wells steadily. The resulting potential shows with being the lattice spacing of the long lattice. The structure of such potential is dependent on both the relative strength of two lattices and the relative phase . When the phase is not equal to ( is an integer), all the double wells are biased with a non-zero tilt between the subsites. In addition, the center of each double well can shift a period of short lattice via changing n by an odd number, which is illustrated in Figure 1 (b).
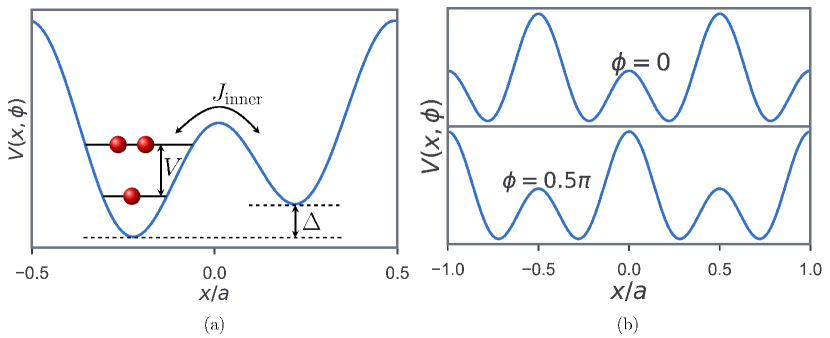
Subsites in each double well are separated by a low barrier, while neighbour double wells are separated by a high barrier. We denote corresponding tunneling strengths as and respectively. As is much smaller than , the hopping event between different double wells can be ignored and the movement of atoms is restricted in each double well. In this case, for two-level bosonic atoms, their dynamics in double wells can be described well with a two-site mode Bose-Hubbard Hamiltonian characterized with , and as follows.
(1) | ||||
Here, denotes right(left) subsite, () is the creation(annihilation) operator for the boson on site i with the inner state , and is the number operator. We consider a non-biased double-well system starting with unit filling—one atom per site. Note that the chemical potential is fixed here for unit filling and thus has no effect on the dynamics, and it is ignored in Eq. (1). In the limit , the large prevents multiple occupation, so that the system would evolve in a subspace consists of four basis states labeled by inner states of the bosons , , and . By using perturbation theory, the above model in this subspace is equivalent to the isotropic Heisenberg Hamiltonian [48]:
(2) |
where , , are Pauli operators on subsite , and is the superexchange coupling between subsites.
In the remaining of this work, we denote spin configuration and as and . Initialized at and driven by this effective Hamiltonian, the state of the system can oscillate between and with a period of while global phase is also recovered, named the superexchange process. Taking an evolution time of , the product states and are prepared into two-qubit maximally entangled states and respectively while and remain unchanged due to the high energy gap of . The effective unitary transformation corresponds to a gate operation, i.e.,
(3) |
with respect to the basis , , , . Moreover, with an evolution time of , one can realize the corresponding gate. In particular, when , the infidelity of the operation caused by the tunneling between neighbour double wells would be smaller than .
For the current gate generation scheme, due to the large ratio , the effective is far less than . In addition, such high requires a deep potential in each site thus leads to a small . As a result, the period of the superexchange process would be long, e.g, tens of milliseconds for 87Rb atoms in superlattice [22, 24], which would aggravate the decoherence effect. To ease this problem, an alternative and faster gate generation scheme can be adopted here [49], which is performed with a small . Instead of introducing the large energy ratio, this scheme utilizes the coherent competition of superexchange and atom tunneling to decrease the component with double occupation in state. As is set to be a finite value , the undesired component can be eliminated completely at specific time intervals. In particular, with an evolution time of , such elimination leads to a fast gate realization with high fidelity.
Besides, the spin-dependent effect further improves the ability to manipulate the atoms in optical lattices. By adding circular polarization components into one lattice light field of superlattice [45, 50], the tilt can be different for different inner states. Therefore, the energy gap between inner states on right subsite would be different from that on the left which induce two applications here. One is to spin flip atoms in one subsite of every double well without affecting atoms in the other subsite, enabling the state transfer between the four basis states mentioned above [45]. The other is to generate a relative phase between and and then transfer the states , to the standard Bell states , [24], respectively.
III.2 Entanglement generation protocol
Our protocol is expected to be performed on one-dimensional atomic chains along X direction with a short lattice isolating the atoms. On each site, the filling is initialized into unit through the SF-MI phase transition. Assuming the inner state of atoms are prepared into and taking 10-qubit system as example, the protocol can be divided into the following steps which are illustrated in Fig. 2.
-
1.
Turn on the spin-dependent superlattice [50] by ramping up a spin-dependent long lattice along X. With selective spin-flip by coupled field, the Néel state is prepared:
(4) -
2.
Turn off the spin-dependent effect and perform gate on the atom pairs in each double well,
(5) -
3.
Turn on the spin-dependent effect again and introduce a relative phase on . As a result, the state of the system is the tensor product of five Bell states,
(6) -
4.
Change the relative phase by to trap the atom pair in different double wells.
-
5.
Perform gates on the atom pairs in each double well, the final state is
(7)
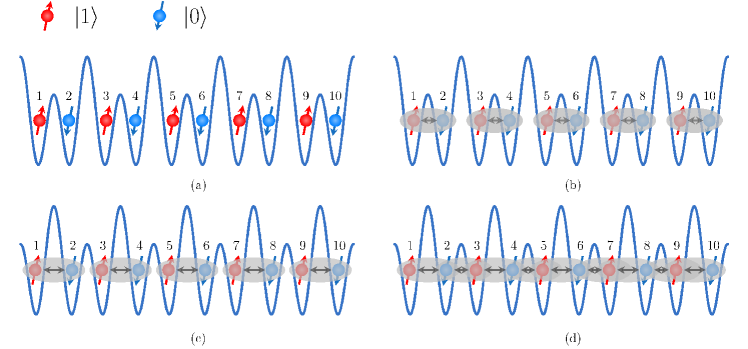
During the entire process atomic motions along other directions are frozen by deep lattice potentials. Finally, one-dimensional entangled systems are generated. The gate operations entangle every pair of neighbour atoms so that the target state is GME by itself. As the gate operation is not present, the depth of each site should be deep enough to avoid any undesirable hopping events.
The target state only owns non-zero projection on a few bases whose total spin numbers are zero, and the magnetic field fluctuation can only introduce a global phase. Consequently, our target can be robust to the magnetic field fluctuation, and thus has longer coherence time, compared to for example the GHZ state. In this basis collection, each basis can find another basis whose spin on each qubit is anti-parallel to its own, e.g. and , and the projections on each pair of bases show same modules but different arguments.
According to the definition of GME [2], it is inferred that a pure state whose subsystems are all mixed states must be a GME state. Based on this, the purity of all subsystems of the target state in the case of 10-qubit are calculated and found to be less than 1, which verifies that this 10-qubit target state possesses GME. This conclusion can be extended to the target state with a different number of qubits.
We remark that Ref. [51] also studies the generation of entanglement by in the cold atom system. The state generated there is like a GHZ state, which needs much more gate operations, compared to the cluster-like state here. In addition, there is no valid method given there to verify the entanglement.
In the following sections, we exhibit theoretical methods to detect the multipartite entanglement in the cold atom system. The prepared state shown in the Sec. III.2 is generated by parallel gates and thus not a stabilizer state, such as the GHZ state and the cluster state. This fact makes the entanglement detection task challenging and the methods proposed in literature are not suitable in this scenario.
To overcome this challenge, we propose three complementary methods. The first one is based on the fidelity estimation to the target state to detect the strongest form of entanglement—genuine multipartite entanglement. By evaluating the non-Pauli stabilizer after the entangling gate evolution, the method only needs constant number of measurement setting with respect to the system size to lower bound the fidelity. To further release the experiment efforts, the second method adopts homogeneous measurements, with only two measurement settings. Thus it is very efficient to realize and can tell whether the prepared state is separable or not considering any bipartition of the whole system. The third one is more intuitional but can reveal GME with less experiment requirements. In particular, we indirectly estimate the fidelity between the prepared state and the target state by evolving the entangling process forward and backward.
All these methods need the spin measurement on single atom, however the fluorescence imaging used in high-resolution experiments can not resolve different spins. As the state we consider here only has one atom on each site, it could be done by measuring the atom distribution after removing one spin component in which the occupied site and unoccupied site represent different spins [52] or splitting different spin components with gradient along perpendicular direction [53].
Before showing these three methods, we give some related definitions about multipartite entanglement.
A pure state is (bi-)separable if it is in a tensor product form , where is a bipartition of the qubits in the system. A mixed state is separable if it can be written as a mixing of pure separable states, . Note that each separable state in the summation can have different bipartitions. The separable state set is denoted as . There is another restricted way for the extension to mixed states. A state is -separable, if it is a mixing of pure separable states with a same partition , and we denote the state set as . It is clear that , and can be generated by the convex mixture of all possible .
Definition 1.
An -qubit quantum state is fully entangled, if it is outside of the separable state set for any bipartition,
(8) |
Definition 2.
An -qubit quantum state possesses genuine multipartite entanglement, if it is outside of the separable state set ,
(9) |
Since , GME is a stronger claim than full entanglement. We also remark that the recently demonstrated entanglement in the IBM cloud quantum computing [54] is actually the full entanglement defined here. By Def. 1, for a state with full entanglement, it is possible to prepare it by mixing bi-separable states with different bipartitions [55]. On the other hand, GME describes the strongest form of quantum entanglement, that is, all the qubits in the system are indeed entangled with each other. GME is essential in various multipartite quantum information tasks, such as quantum cryptography [56], quantum nonlocality [57], quantum networks [58, 59], quantum metrology [60] and measurement-based quantum computing [61].
III.3 Entanglement detection based on fidelity estimation
In this section, we show an entanglement detection protocol based on the fidelity value between the prepared state and the target state.
Proposition 1.
The operator can witness genuine multipartite entanglement near ,
(10) |
with for any separable state in .
According to Proposition 1, if the fidelity of the prepared state with the target state , i.e., , exceeds , possesses GME. However, it is generally difficult to evaluate the quantity by the direct projection on , as it is an entangled state.
Alternatively, one needs to decompose the density operator into the summation of many local measurements in the form , which is easier to implement in experiments. Here is Hermitian operator of the i-th qubit. The number of local measurements characterizes the experiment effort to estimate the fidelity.
Here, in order to reduce the measurement effort, instead of direct decomposing , we give a lower bound of the fidelity by using the stabilizer-like operator for the non-stabilizer state .
Proposition 2.
For the target state and its independent stabilizers , the following inequality holds,
(11) |
where indicates that is positive semidefinite. The stabilizer is determined by the evolution of gates with , where
(12) |
for , are the stabilizers for the Bell pairs.
Due to is not a Clifford gate, the corresponding stabilizer is not in a tensor product of Pauli operators, but the summation of them. One can directly get by the evolution of the parallel on . Due to the locality of the evolution, can only be transformed by the gate with overlapping support. For example,
(13) |
where each new stabilizer is the summation of four Pauli operator. For the stabilizers in the bulk of the 1-D chain, there are two on them. Take and as example, and are performed on them. As a result, the corresponding stabilizers are:
(14) |
Both are the linear combinations of 16 Pauli tensors. For other stabilizers, similar forms could be obtained in this way.
In total, the number of these Pauli tensors is almost which scales linearly with the qubit number. In fact, the measurement effort can be further reduced, as several Pauli tensors can be grouped and measured in one local measurement setting (LMS) simultaneously. For the Pauli tensors from , we introduce the following expression for the example case ,
(15) | ||||
where one can periodically select one of the three Pauli tensors in every brace to construct one LMS, such that these LMSs cover all the possible Pauli operators from . That is, select same tensor in (2,3) and (6,7) and the same Pauli operator in (4,5) and (8,9). Thus, only 9 LMSs are needed here. Following the same way, we can also find another 9 LMSs for the Pauli operators from . As a result, totally only a constant number of LMSs is needed to obtain all the expectation values of the stabilizers.
To evaluate the robustness of our witness, we apply the white noise model , and it shows that the noise tolerance is . As , it equals . In fact, by utilizing the trade-off between the robustness and the measure budget [62, 63], one can enhance the noise tolerance of the witness further. In Ref. [64], some of us further generalize the witness here by utilizing more local measurement settings, and the witnesses constructed there would be more suitable to realize in other quantum systems, such as superconducting-qubit. In particular, a numerical algorithm is developed in Ref. [64] to search for the witness with the optimal noise tolerance under given measurement settings. For instance, one can reach the noise tolerance with about settings.
III.4 Entanglement detection with homogeneous measurements
The entanglement witness shown in Sec. III.3 based on the fidelity estimation can detect GME, with a few number of LMSs. However, in each of the LMS, the Pauli operators may be not the same, such as . This kind of inhomogeoneus measurement needs additional local basis rotation, which is challenging for the current cold atom system.
In this section, we reduce the measurement efforts further by only considering the homogenous measurement, say . In particular, we only need two LMSs, and . Note that and are symmetric for our generation, thus we only need to measure one of them. Detailed illustration on this point is given in Method.
Instead of detecting GME, the protocol here aims to detect the full entanglement property defined in Eq. (8), i.e., not separable with respective to any bipartition. First, let us show the following Lemma which plays an important role of the detection.
Lemma 1.
For a -qubit quantum state with being even, if it is -separable, with and the number of qubits contained in and being odd, the following inequality holds,
(16) |
where is the expectation value.
The proof of Lemma is based on the anticommutative relation [65, 66] on subsystems and respectively. As , it becomes to the common criterion for the Bell state. As a result, the violation of the bound in Eq. (16) indicates that the underlying even-qubit state is non-separable regarding any odd-odd bipartitions.
Second, we give the following observation which shows the relation of the entanglement property of the state and its reduced density matrix (RDM).
Observation 1.
Suppose a quantum state is created from by local operation and classical communications(LOCC) with respective to the bipartiton , i.e.,
(17) |
If is separable for the bipartition , is also separable for the bipartition ; in other words, if is entangled for , is also entangled for .
The observation holds as LOCC can not create entanglement. Specifically, the partial trace operation is an example of LOCC, where are subsystems of respectively.
By Definition 1, an -qubit quantum state is fully entangled, if it cannot been written in the following form
(18) |
for all possible bipartitions of the whole system. In the following, we employ Lemma 1 and Observation 1 time and time again on RDMs of the 1-D prepared state, and finally certify its full entanglement. The RDM of the prepared state , for example, on the first two qubits, is denoted by . Note that the given expectation values are from the perfect target state. The practical value in the experiment may deviate from it, but can still reveal the entanglement property if the deviation is not too large. Detailed noise analysis are shown in Method.
To certify the full entanglement, we prove it by contradiction. Specifically, we first assume that the state can be written as right hand side of Eq. (18) for some , and then show that all the qubit are either in or in , which is actually not separable and leads to the contradiction.
Here we take for simplicity, and it can be directly generalized to any even qubit number . The procedure is listed as follows.
-
1.
For the RDM , one has
(19) with for the target state. It indicates that is non-separable on account of Lemma 1. Thus qubits should both be contained in or , otherwise it violates Observation 1. Without loss of generality, we assume are in . Similarly, also satisfies the inequality in Eq. (19), and thus are all in .
-
2.
In fact, one can not proceed along the chain, since the RDMs are not entangled. Thus we consider correlations involving more qubits. For the RDM , one has
(20) with for the target state. It indicates that is non-separable for the bipartition due to Lemma 1. Thus qubits should all be contained in , due to Observation 1 and Step 1.
-
3.
Same as Step 1, by applying Eq. (19) for and , one can proceed to conclude that qubits should all be contained in or . Due to the result got in Step 2, we can arrive at the result that all the qubits are contained in . This is contradict to the separable state on the right hand side of Eq. (18). Thus the prepared state owns full entanglement.
III.5 Entanglement detection with reverse evolution
Compared with the previous two entanglement detection protocols, the third one is more intuitional and aims to reveal GME. As shown in Sec. III.3, it is not easy to obtain the fidelity to the target non-stabilizer state. The first method should use several non-homogenous LMSs to lower bound the fidelity.
In this section, we indirectly estimate the fidelity between the prepared state and the target state by evolving the entangling process backwards. Since the fidelity to both the Bell state and product state can be measured in the experiment, we can indirectly estimate the fidelity to and certify GME according to Lemma 1. The reverse evolution follows the state preparation process shown in Sec. III.2.
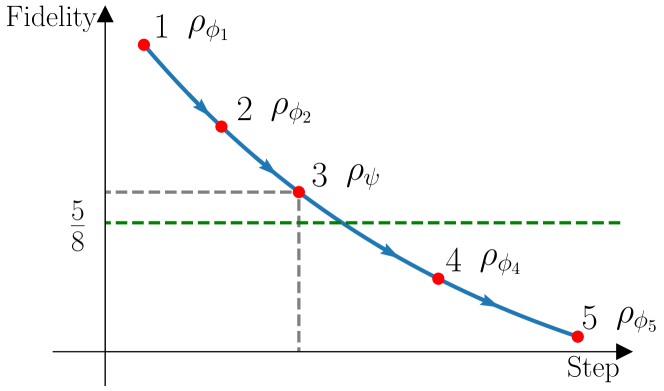
-
•
Operate gates parallelly on the qubit pairs .
(21) -
•
Adjust the relative phase between and in each of the Bell pairs in .
(22) -
•
Operate gates parallelly on the qubit pairs .
(23)
In the above reverse protocol, the state is evolved backwards finally to the initial product state. Note that here we list the perfect reverse evolution, that is, and shown in the generation protocol in Sec. III.2. The practical states actually deviate from them due to noises, denoted by for , and the fidelity decreases due to the imperfection of the underlying gates.
One can estimate the fidelity to product states with the measurement setting, entangled-pair states with and , and Bell-pair states with and . In Fig. 3, we show an illustration. By fitting the fidelity values from the experiment, one can indirectly estimate the fidelity of the prepared state to the target state , which can be compared with the theoretical bound for GME, say , denoted by the dotted horizon line in the figure. Note that , i.e., it takes three times of evolution time, and one may needs to consider this point in the fitting. We remark that this entanglement detection method is intuitive, and one could make it more rigorous by assuming more specific noise models.
IV Discussion
In this work, we propose an experimental scheme to generate and characterize large-scale entanglement of cold atoms confined in the optical superlattice. The generation scheme utilizes the entangling gate induced by the superexchange interaction, and is robust to decoherence. To characterize the entanglement, we propose several complementary methods considering the experimental implementation feasibility. Moreover, it is straightforward to generate our scheme to the high dimension scenarios, and it is also interesting to construct some efficient entanglement verification tools for them. In summary, our entanglement generation and verification protocols are well tailored for the cold atom system, and lay the foundation for the further applications, such as measurement-based quantum computing.
V Methods
V.1 Proof of Proposition 1
Proof.
To prove that Eq. (10) is a legal GME witness, we need to show that the maximal fidelity between the separable state and our target state is upper bounded by , that is
(24) |
By the convexity of , we can reduce the maximization to the pure separable state
(25) | ||||
where we maximize over from a given bipartition and also all possible bipartitions. The second line is due to the fact that the maximization result over is just the square of the largest Schmidt coefficient of with respective to [67], where the Schmidt decomposition shows and .
In fact, we only need to consider the bipartitions where contains the first and contains the last qubits on the 1-D chain. That is, there is only one boundary between and . Other choices with more boundaries can lead to a smaller . Consider the one boundary scenario, there are two types of bipartations depending on that the boundary is between or , as shown in Fig. 4.
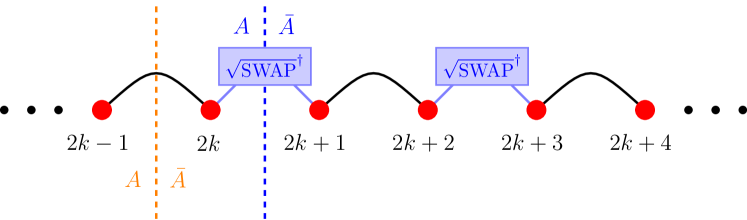
Based on the causality of the quantum gate and the fact that quantum gates inside the subsystem and do not change the Schmidt number, we can determine for both of cases. For the case, , since we can ignore the latter and the entanglement spectrum is just the same with that of the Bell state.
For the case, we just need to focus on the four-qubit state after the ignorance of the other distant gates, that is,
(26) |
Here for denotation simplicity we take without loss of generality, and . By calculation one finds that the reduced density matrix of the first two-qubit is
(27) |
i.e., the mixture of the Bell state and the maximal mixed state. As a result, is a Bell-diagonal state and thus its four eigenvalues on the Bell basis is with . In summary, we prove that . ∎
V.2 Proof of Proposition 2
Proof.
We first show that stabilizes the target by definition. Before the final layer of gates, the state is the product of a few of Bell pairs, denoted by , which is stabilized by defined in Eq. (12). That is, . Thus, one has .
Then we prove the inequality in Eq. (11). We remark that Eq. (11) holds for any generalized stabilizer state. The independent stabilizers commute with each other and their common eigenstates together determine an orthogonal basis denoted by . Here with each taking or and . It is clear our target state . It is clear that both operators on the left and right of Eq. (11) are diagonal in this basis, thus we can prove it by checking for the matrix elements for every . For , one has . For which contains only one zero, such as , one has . For the containing more zeros, one can prove the inequality similarly. ∎
V.3 Symmetry of the target state
Remember that in principle we should apply three measurement settings to detect the full entanglement in Sec. III.4. Here we show rigorously as follows that one does not need to worry about the direction on the plane in every measurement. In fact, one only needs to measure by the symmetry of the target state.
In the experiment, actually there is no reference pulse to decide the direction of the measurement, that is, one chooses a random angle for the measurement. Note that there is a corresponding unitary (rotation around ),
(28) |
such that .
As a result, suppose there is a standard direction, the actual measurement shows,
(29) | ||||
where is the prepared state in the experiment, and . In other words, measuring the state in random direction is equivalent to “twirling” the state, and the resulting state is symmetric with respective to the measurement direction.
(30) |
Specifically, and , and one also has
(31) |
since commutes with defined in Eq. (28).
It is not hard to see that the entanglement of is not stronger than , since is obtained from using LOCC, that is, the twirling can not increase the entanglement. Thus if one can detect the entanglement property of , this property should also holds for .
On the other hand, the target state is a symmetric state on plane, i.e., . In fact, one can write the rotation unitary as
(32) |
Since our target state only has non-zero projection on the computational bases whose total spin is zero, i.e., half number of 0 and 1, the rotation unitary introduce the same phase for these bases, and the state is unchanged.
V.4 Proof of Lemma 1
Proof.
Without loss of generality, we assume that the subsystem contains the first qubits, and , are both odd numbers. Since the left hand of Eq. (16) is a convex function of the state, thus we only need to consider the pure separable state in the form , and the expectation value can be written apart as,
(33) | ||||
where the second line is due to Cauchy-Schwarz inequality. Since is an odd number, one can check that and anticommute with each other, and thus [65, 66]. Similarly one has . As a result, we finish the proof. ∎
V.5 Proof of Observation 1
Proof.
The Observation is right by the definition of LOCC. Here we show the case for the partial trace operation by contradiction. Suppose is separable. The partial trace and , such that is still separable, which contradicts with the assumption that it is entangled. ∎
V.6 Influence from Operation Errors
The fidelity of the state creation is affected by the error of each operation, caused by the decoherence effect and noises. For the large-scale entangled state, the degradation of fidelity and also the entanglement detection is more apparent compared to few-body states. In the meantime, the read-out error also affects the entanglement detection. As a result, it is meaningful to analyze the influence of various errors in the experimental implementation.
Here we consider the errors in the following operations: the initial preparation of Néel state; the final projective measurement of spins; and the fidelity of the intermediate quantum gates. We show their influences on the entanglement detection of a ten-qubit system, i.e., , by using the witness method in Sec. III.4, which is more practical for experiments. As shown in the procedure of entanglement detection in Sec. III.4, we should measure the witness value in Eq. (16) for a few of reduced density matrices of subsystems and also the whole system. Here we take the subsystem as
(34) | ||||
which contain qubits.
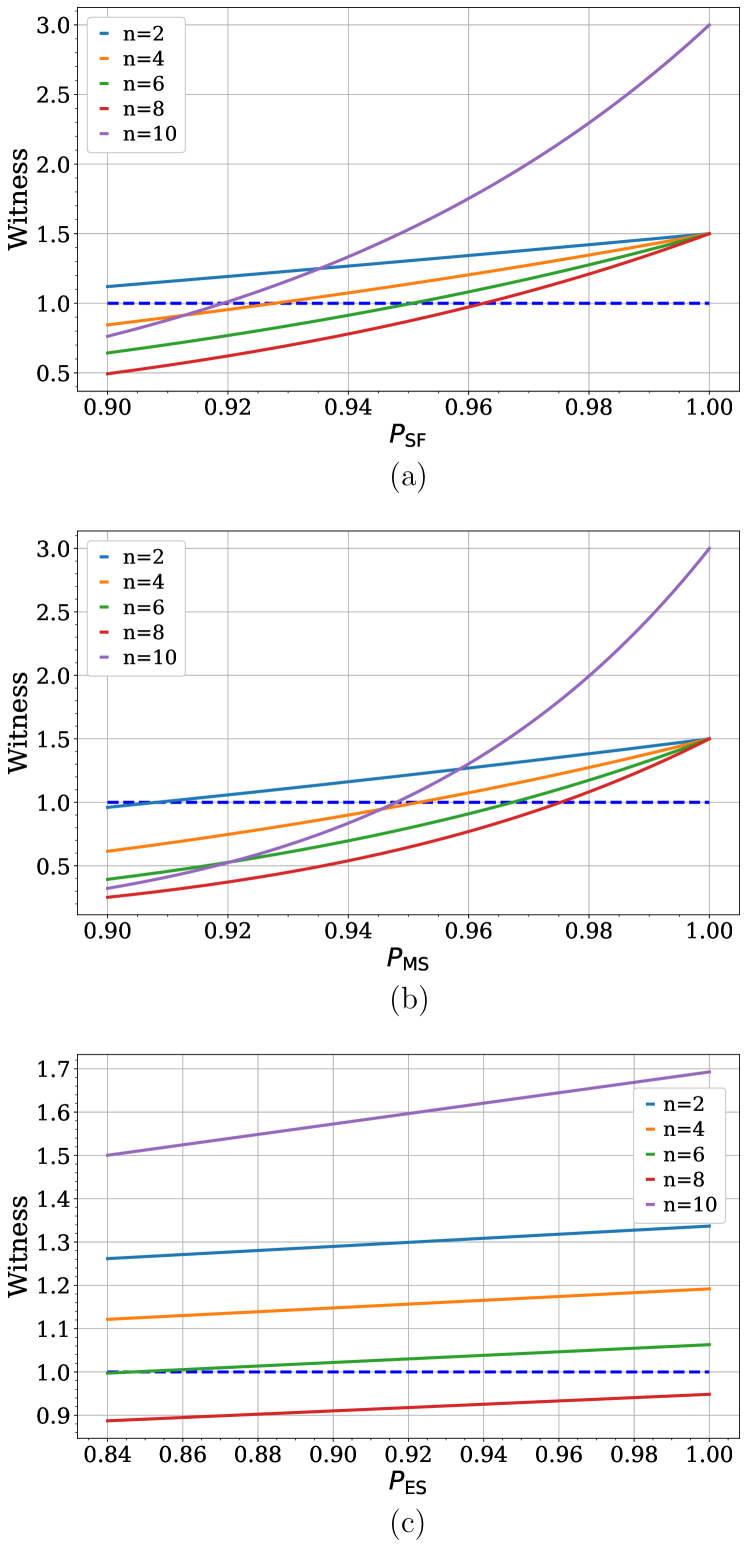
In the first step of Sec. III.2, starting from the all-zero state , one should flip the spins on the odd sites to prepare the Néel state in Eq. (4). There is the probability of a non-flip for the odd site, and also the probability of a wrong flip for the even site. For simplicity, we assume both error probabilities are equal, and denote the probability of the correct flip as for each site. In this case, the system is initially prepared into a mixture of different product states weighted with corresponding probabilities. In Fig. 5 (a), we plot the witness values of Eq. (16) for different subsystem size with respective to . Similarly, in the final measurement, the probability to measure the spin of single atom correctly is denoted as . That is, there is probability to recognize as or vice versa. The witness value with respective to is shown in Fig. 5 (b). From these two figures, we found that the witness values are the same for the subsystem with the same qubit number, for example, case with and . When there is no error, say and , all the witness values return for all the subsystems, except the whole system with the value . One can see that even though there is some error, the witness can detect the entanglement as the value is larger than . For larger subsystems, the values decay faster with and .
Similar as the six-qubit case discussed in Sec. III.4, here for total system size , one only needs to measure the witness for to verify the full entanglement. As a result, the result of the subsystems with at most qubits decides the lower bound of the operation fidelity. According to Fig. 5 (a) and (b), the bounds are 0.95 and 0.97 for and respectively.
At last, in Fig. 5 (c), we study the influence of the fidelity of the entangling step between the above two steps by taking and . Here we assume that this operation has a probability to be performed perfectly while it contributes zero to the witness with probability , that is, essentially outputs a maximal mixed state. As shown in Fig. 5 (c), the full entanglement could be verified when the fidelity of entangling step exceeds .
VI CODE AVAILABILITY
The code that supports the findings of this study are available from the corresponding author upon reasonable request.
VII DATA AVAILABILITY
Data sharing is not applicable to this article as no data sets were generated or analyzed during the current study.
VIII Acknowledgments
This work was supported by the National Natural Science Foundation of China (NNSFC) No. 12125409, the Anhui Initiative in Quantum Information Technologies, and the Chinese Academy of Sciences. X. Ma acknowledges the support by the NNSFC No. 11875173 and No. 12174216 and the National Key Research and Development Program of China No. 2019QY0702 and No. 2017YFA0303903.
IX COMPETING INTERESTS
The authors declare that there are no competing interests.
X AUTHOR CONTRIBUTIONS
All authors contributed to the theory, numerical analysis, and writing up the manuscript. Y. Z. and B. X. contributed equally to this work.
XI References
References
- Nielsen and Chuang [2011] Michael A. Nielsen and Isaac L. Chuang, Quantum Computation and Quantum Information: 10th Anniversary Edition, 10th ed. (Cambridge University Press, New York, NY, USA, 2011).
- Horodecki et al. [2009] Ryszard Horodecki, Paweł Horodecki, Michał Horodecki, and Karol Horodecki, “Quantum entanglement,” Rev. Mod. Phys. 81, 865–942 (2009).
- Monz et al. [2011] Thomas Monz et al., “14-qubit entanglement: Creation and coherence,” Phys. Rev. Lett. 106 (2011).
- Nigg et al. [2014] D. Nigg et al., “Quantum computations on a topologically encoded qubit,” Science 345, 302–305 (2014).
- Friis et al. [2018] Nicolai Friis et al., “Observation of entangled states of a fully controlled 20-qubit system,” Phys. Rev. X 8 (2018).
- Wang et al. [2018a] Xi-Lin Wang et al., “18-qubit entanglement with six photons’ three degrees of freedom,” Phys. Rev. Lett. 120 (2018a).
- Zhong et al. [2018] Han-Sen Zhong et al., “12-photon entanglement and scalable scattershot boson sampling with optimal entangled-photon pairs from parametric down-conversion,” Phys. Rev. Lett. 121 (2018).
- Omran et al. [2019] A. Omran et al., “Generation and manipulation of schrödinger cat states in rydberg atom arrays,” Science 365, 570–574 (2019).
- Gong et al. [2019] Ming Gong et al., “Genuine 12-qubit entanglement on a superconducting quantum processor,” Phys. Rev. Lett. 122 (2019).
- Wei et al. [2020] Ken X. Wei et al., “Verifying multipartite entangled greenberger-horne-zeilinger states via multiple quantum coherences,” Phys. Rev. A 101 (2020).
- Song et al. [2019] Chao Song et al., “Generation of multicomponent atomic schrödinger cat states of up to 20 qubits,” Science 365, 574–577 (2019).
- Zhang et al. [2017] J. Zhang et al., “Observation of a many-body dynamical phase transition with a 53-qubit quantum simulator,” Nature 551, 601–604 (2017).
- Arute et al. [2019] Frank Arute et al., “Quantum supremacy using a programmable superconducting processor,” Nature 574, 505–510 (2019).
- Preskill [2018] John Preskill, “Quantum Computing in the NISQ era and beyond,” Quantum 2, 79 (2018).
- Bloch et al. [2012] Immanuel Bloch, Jean Dalibard, and Sylvain Nascimbène, “Quantum simulations with ultracold quantum gases,” Nat. Phys. 8, 267–276 (2012).
- Jaksch et al. [1998] D. Jaksch, C. Bruder, J. I. Cirac, C. W. Gardiner, and P. Zoller, “Cold bosonic atoms in optical lattices,” Phys. Rev. Lett. 81, 3108–3111 (1998).
- Greiner et al. [2002] Markus Greiner, Olaf Mandel, Tilman Esslinger, Theodor W. Hänsch, and Immanuel Bloch, “Quantum phase transition from a superfluid to a mott insulator in a gas of ultracold atoms,” Nature 415, 39–44 (2002).
- Jaksch et al. [1999] D. Jaksch, H.-J. Briegel, J. I. Cirac, C. W. Gardiner, and P. Zoller, “Entanglement of atoms via cold controlled collisions,” Phys. Rev. Lett. 82, 1975–1978 (1999).
- Mandel et al. [2003] Olaf Mandel, Markus Greiner, Artur Widera, Tim Rom, Theodor W. Hänsch, and Immanuel Bloch, “Controlled collisions for multi-particle entanglement of optically trapped atoms,” Nature 425, 937–940 (2003).
- Raussendorf and Briegel [2001a] Robert Raussendorf and Hans J. Briegel, “A one-way quantum computer,” Phys. Rev. Lett. 86, 5188–5191 (2001a).
- Raussendorf et al. [2003] Robert Raussendorf, Daniel E. Browne, and Hans J. Briegel, “Measurement-based quantum computation on cluster states,” Phys. Rev. A 68, 022312 (2003).
- Trotzky et al. [2008] S. Trotzky et al., “Time-resolved observation and control of superexchange interactions with ultracold atoms in optical lattices,” Science 319, 295–299 (2008).
- Anderlini et al. [2007] Marco Anderlini, Patricia J. Lee, Benjamin L. Brown, Jennifer Sebby-Strabley, William D. Phillips, and J. V. Porto, “Controlled exchange interaction between pairs of neutral atoms in an optical lattice,” Nature 448, 452–456 (2007).
- Dai et al. [2016] Han-Ning Dai et al., “Generation and detection of atomic spin entanglement in optical lattices,” Nat. Phys. 12, 783–787 (2016).
- Scholl et al. [2021] Pascal Scholl et al., “Quantum simulation of 2D antiferromagnets with hundreds of Rydberg atoms,” Nature 595, 233–238 (2021).
- Scholl et al. [2022] P. Scholl et al., “Microwave engineering of programmable hamiltonians in arrays of rydberg atoms,” PRX Quantum 3, 020303 (2022).
- Weitenberg et al. [2011] Christof Weitenberg et al., “Single-spin addressing in an atomic mott insulator,” Nature 471, 319–324 (2011).
- Bakr et al. [2009] Waseem S. Bakr, Jonathon I. Gillen, Amy Peng, Simon Fölling, and Markus Greiner, “A quantum gas microscope for detecting single atoms in a hubbard-regime optical lattice,” Nature 462, 74–77 (2009).
- Sherson et al. [2010] Jacob F. Sherson, Christof Weitenberg, Manuel Endres, Marc Cheneau, Immanuel Bloch, and Stefan Kuhr, “Single-atom-resolved fluorescence imaging of an atomic mott insulator,” Nature 467, 68–72 (2010).
- Parsons et al. [2015] Maxwell F. Parsons, Florian Huber, Anton Mazurenko, Christie S. Chiu, Widagdo Setiawan, Katherine Wooley-Brown, Sebastian Blatt, and Markus Greiner, “Site-resolved imaging of FermionicLi6in an optical lattice,” Phys. Rev. Lett. 114 (2015).
- Cheuk et al. [2015] Lawrence W. Cheuk et al., “Quantum-gas microscope for fermionic atoms,” Phys. Rev. Lett. 114 (2015).
- Haller et al. [2015] Elmar Haller et al., “Single-atom imaging of fermions in a quantum-gas microscope,” Nat. Phys. 11, 738–742 (2015).
- Vogel and Risken [1989] K. Vogel and H. Risken, “Determination of quasiprobability distributions in terms of probability distributions for the rotated quadrature phase,” Phys. Rev. A 40, 2847–2849 (1989).
- Paris and Rehacek [2004] M. Paris and J. (eds) Rehacek, “Quantum state estimation,” Lect. Notes Phys. (2004).
- Terhal [2001] Barbara M. Terhal, “A family of indecomposable positive linear maps based on entangled quantum states,” Linear Algebra Appl. 323, 61 – 73 (2001).
- Guhne and Toth [2009] Otfried Guhne and Geza Toth, “Entanglement detection,” Phys. Rep. 474, 1 – 75 (2009).
- Friis et al. [2019] Nicolai Friis, Giuseppe Vitagliano, Mehul Malik, and Marcus Huber, “Entanglement certification from theory to experiment,” Nat. Rev. Phys. 1, 72–87 (2019).
- Tóth et al. [2009] Géza Tóth, Witlef Wieczorek, Roland Krischek, Nikolai Kiesel, Patrick Michelberger, and Harald Weinfurter, “Practical methods for witnessing genuine multi-qubit entanglement in the vicinity of symmetric states,” New J. Phys. 11, 083002 (2009).
- Tóth et al. [2010] G. Tóth, W. Wieczorek, D. Gross, R. Krischek, C. Schwemmer, and H. Weinfurter, “Permutationally invariant quantum tomography,” Phys. Rev. Lett. 105, 250403 (2010).
- Zhou et al. [2019a] You Zhou, Chenghao Guo, and Xiongfeng Ma, “Decomposition of a symmetric multipartite observable,” Phys. Rev. A 99, 052324 (2019a).
- Tóth and Gühne [2005a] Géza Tóth and Otfried Gühne, “Detecting genuine multipartite entanglement with two local measurements,” Phys. Rev. Lett. 94, 060501 (2005a).
- Knips et al. [2016] Lukas Knips, Christian Schwemmer, Nico Klein, Marcin Wieśniak, and Harald Weinfurter, “Multipartite entanglement detection with minimal effort,” Phys. Rev. Lett. 117, 210504 (2016).
- Zhou et al. [2019b] You Zhou, Qi Zhao, Xiao Yuan, and Xiongfeng Ma, “Detecting multipartite entanglement structure with minimal resources,” npj Quantum Inf. 5, 83 (2019b).
- Sebby-Strabley et al. [2006] J. Sebby-Strabley, M. Anderlini, P. S. Jessen, and J. V. Porto, “Lattice of double wells for manipulating pairs of cold atoms,” Phys. Rev. A 73 (2006).
- Lee et al. [2007] P. Lee, M. Anderlini, B. Brown, J. Sebby-Strabley, W. Phillips, and J. Porto, “Sublattice addressing and spin-dependent motion of atoms in a double-well lattice,” Phys. Rev. Lett. 99 (2007).
- Trotzky et al. [2010] Stefan Trotzky, Yu-Ao Chen, Ute Schnorrberger, Patrick Cheinet, and Immanuel Bloch, “Controlling and detecting spin correlations of ultracold atoms in optical lattices,” Phys. Rev. Lett. 105 (2010).
- Lohse et al. [2015] M. Lohse, C. Schweizer, O. Zilberberg, M. Aidelsburger, and I. Bloch, “A thouless quantum pump with ultracold bosonic atoms in an optical superlattice,” Nat. Phys. 12, 350–354 (2015).
- Duan et al. [2003] L.-M. Duan, E. Demler, and M. D. Lukin, “Controlling spin exchange interactions of ultracold atoms in optical lattices,” Phys. Rev. Lett. 91 (2003).
- Yang et al. [2020] Bing Yang et al., “Cooling and entangling ultracold atoms in optical lattices,” Science , eaaz6801 (2020).
- Yang et al. [2017] Bing Yang, Han-Ning Dai, Hui Sun, Andreas Reingruber, Zhen-Sheng Yuan, and Jian-Wei Pan, “Spin-dependent optical superlattice,” Phys. Rev. A 96 (2017).
- Vaucher et al. [2008] B Vaucher, A Nunnenkamp, and D Jaksch, “Creation of resilient entangled states and a resource for measurement-based quantum computation with optical superlattices,” New J. Phys. 10, 023005 (2008).
- Parsons et al. [2016] Maxwell F. Parsons, Anton Mazurenko, Christie S. Chiu, Geoffrey Ji, Daniel Greif, and Markus Greiner, “Site-resolved measurement of the spin-correlation function in the fermi-hubbard model,” Science 353, 1253–1256 (2016).
- Boll et al. [2016] Martin Boll et al., “Spin- and density-resolved microscopy of antiferromagnetic correlations in fermi-hubbard chains,” Science 353, 1257–1260 (2016).
- Wang et al. [2018b] Yuanhao Wang, Ying Li, Zhang-qi Yin, and Bei Zeng, “16-qubit ibm universal quantum computer can be fully entangled,” npj Quantum Inf. 4, 46 (2018b).
- Gühne and Tóth [2009] Otfried Gühne and Géza Tóth, “Entanglement detection,” Physics Reports 474, 1–75 (2009).
- Chen and Lo [2007] Kai Chen and Hoi-Kwong Lo, “Multi-partite quantum cryptographic protocols with noisy GHZ states,” Quantum Inf. Comput. 7, 689–715 (2007).
- Brunner et al. [2014] Nicolas Brunner, Daniel Cavalcanti, Stefano Pironio, Valerio Scarani, and Stephanie Wehner, “Bell nonlocality,” Reviews of Modern Physics 86, 419 (2014).
- Wehner et al. [2018] Stephanie Wehner, David Elkouss, and Ronald Hanson, “Quantum internet: A vision for the road ahead,” Science 362, eaam9288 (2018).
- Perseguers et al. [2013] S Perseguers, G J Lapeyre, D Cavalcanti, M Lewenstein, and A Acín, “Distribution of entanglement in large-scale quantum networks,” Rep. Prog. Phys. 76, 096001 (2013).
- Giovannetti et al. [2011] Vittorio Giovannetti, Seth Lloyd, and Lorenzo Maccone, “Advances in quantum metrology,” Nat. Photonics 5, 222–229 (2011).
- Raussendorf and Briegel [2001b] Robert Raussendorf and Hans J. Briegel, “A one-way quantum computer,” Phys. Rev. Lett. 86, 5188–5191 (2001b).
- Gühne et al. [2007] Otfried Gühne, Chao-Yang Lu, Wei-Bo Gao, and Jian-Wei Pan, “Toolbox for entanglement detection and fidelity estimation,” Phys. Rev. A 76, 030305(R) (2007).
- Zhao et al. [2019] Qi Zhao, Gerui Wang, Xiao Yuan, and Xiongfeng Ma, “Efficient and robust detection of multipartite greenberger-horne-zeilinger-like states,” Phys. Rev. A 99, 052349 (2019).
- Zhang et al. [2021] Yihong Zhang, Yifan Tang, You Zhou, and Xiongfeng Ma, “Efficient entanglement generation and detection of generalized stabilizer states,” Phys. Rev. A 103, 052426 (2021).
- Tóth and Gühne [2005b] Géza Tóth and Otfried Gühne, “Entanglement detection in the stabilizer formalism,” Phys. Rev. A 72, 022340 (2005b).
- Asadian et al. [2016] Ali Asadian, Paul Erker, Marcus Huber, and Claude Klöckl, “Heisenberg-weyl observables: Bloch vectors in phase space,” Phys. Rev. A 94, 010301 (2016).
- Bourennane et al. [2004] Mohamed Bourennane et al., “Experimental detection of multipartite entanglement using witness operators,” Phys. Rev. Lett. 92, 087902 (2004).